
- •Contents
- •Contributors
- •Brain Tumor Imaging
- •1 Introduction
- •1.1 Overview
- •2 Clinical Management
- •3 Glial Tumors
- •3.1 Focal Glial and Glioneuronal Tumors Versus Diffuse Gliomas
- •3.3 Astrocytomas Versus Oligodendroglial Tumors
- •3.4.1 Diffuse Astrocytoma (WHO Grade II)
- •3.5 Anaplastic Glioma (WHO Grade III)
- •3.5.1 Anaplastic Astrocytoma (WHO Grade III)
- •3.5.3 Gliomatosis Cerebri
- •3.6 Glioblastoma (WHO Grade IV)
- •4 Primary CNS Lymphomas
- •5 Metastatic Tumors of the CNS
- •References
- •MR Imaging of Brain Tumors
- •1 Introduction
- •2 Brain Tumors in Adults
- •2.1 Questions to the Radiologist
- •2.2 Tumor Localization
- •2.3 Tumor Malignancy
- •2.4 Tumor Monitoring
- •2.5 Imaging Protocol
- •Computer Tomography
- •2.6 Case Illustrations
- •3 Pediatric Brain Tumors
- •3.1 Standard MRI
- •3.2 Differential Diagnosis of Common Pediatric Brain Tumors
- •3.3 Early Postoperative Imaging
- •3.4 Meningeal Dissemination
- •References
- •MR Spectroscopic Imaging
- •1 Methods
- •1.1 Introduction to MRS
- •1.2 Summary of Spectroscopic Imaging Techniques Applied in Tumor Diagnostics
- •1.3 Partial Volume Effects Due to Low Resolution
- •1.4 Evaluation of Metabolite Concentrations
- •1.5 Artifacts in Metabolite Maps
- •2 Tumor Metabolism
- •3 Tumor Grading and Heterogeneity
- •3.1 Some Aspects of Differential Diagnosis
- •4 Prognostic Markers
- •5 Treatment Monitoring
- •References
- •MR Perfusion Imaging
- •1 Key Points
- •2 Methods
- •2.1 Exogenous Tracer Methods
- •2.1.1 Dynamic Susceptibility Contrast MRI
- •2.1.2 Dynamic Contrast-Enhanced MRI
- •3 Clinical Application
- •3.1 General Aspects
- •3.3 Differential Diagnosis of Tumors
- •3.4 Tumor Grading and Prognosis
- •3.5 Guidance for Biopsy and Radiation Therapy Planning
- •3.6 Treatment Monitoring
- •References
- •Diffusion-Weighted Methods
- •1 Methods
- •2 Microstructural Changes
- •4 Prognostic Marker
- •5 Treatment Monitoring
- •Conclusion
- •References
- •1 MR Relaxometry Techniques
- •2 Transverse Relaxation Time T2
- •4 Longitudinal Relaxation Time T1
- •6 Cest Method
- •7 CEST Imaging in Brain Tumors
- •References
- •PET Imaging of Brain Tumors
- •1 Introduction
- •2 Methods
- •2.1 18F-2-Fluoro-2-Deoxy-d-Glucose
- •2.2 Radiolabeled Amino Acids
- •2.3 Radiolabeled Nucleoside Analogs
- •2.4 Imaging of Hypoxia
- •2.5 Imaging Angiogenesis
- •2.6 Somatostatin Receptors
- •2.7 Radiolabeled Choline
- •3 Delineation of Tumor Extent, Biopsy Guidance, and Treatment Planning
- •4 Tumor Grading and Prognosis
- •5 Treatment Monitoring
- •7 PET in Patients with Brain Metastasis
- •8 Imaging of Brain Tumors in Children
- •9 Perspectives
- •References
- •1 Treatment of Gliomas and Radiation Therapy Techniques
- •2 Modern Methods and Strategies
- •2.2 3D Conformal Radiation Therapy
- •2.4 Stereotactic Radiosurgery (SRS) and Radiotherapy
- •2.5 Interstitial Brachytherapy
- •2.6 Dose Prescription
- •2.7 Particle Radiation Therapy
- •3 Role of Imaging and Treatment Planning
- •3.1 Computed Tomography (CT)
- •3.2 Magnetic Resonance Imaging (MRI)
- •3.3 Positron Emission Tomography (PET)
- •4 Prognosis
- •Conclusion
- •References
- •1 Why Is Advanced Imaging Indispensable for Modern Glioma Surgery?
- •2 Preoperative Imaging Strategies
- •2.4 Preoperative Imaging of Function and Functional Anatomy
- •2.4.1 Imaging of Functional Cortex
- •2.4.2 Imaging of Subcortical Tracts
- •3 Intraoperative Allocation of Relevant Anatomy
- •Conclusions
- •References
- •Future Methods in Tumor Imaging
- •1 Special Editing Methods in 1H MRS
- •1.1 Measuring Glycine
- •2 Other Nuclei
- •2.1.1 Spatial Resolution
- •2.1.2 Measuring pH
- •2.1.3 Measuring Lipid Metabolism
- •2.1.4 Energy Metabolism
- •References
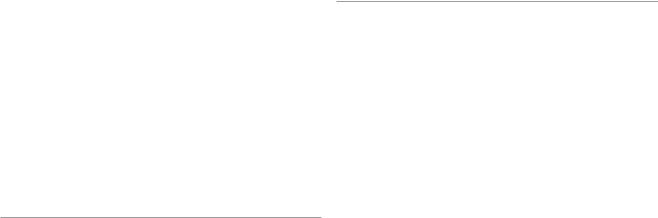
Advanced MR Methods in Differential Diagnosis of Brain Tumors |
115 |
|
|
perfusion measurement may be considered as “hypoxia imaging” (Tóth et al. 2013). Tumor hypoxia is considered as an important motor of malignant transformation and, therefore, increases the tumor’s aggressiveness. It also causes resistance of the tumor to chemotherapy and radiation mediated by the activation of HIF-1α and carbonic anhydrase IX and XII (Harguindey et al. 2009). The indirect measurement of hypoxia by T2′ quantification might provide insight into the pathophysiology of brain tumors and into the effects of therapy (Hattingen et al. 2013; Hoskin et al. 2007). Saitta et al. could show that high-grade tumors revealed lower T2′ values, suggesting a higher degree of hypoxia in these fast growing and proliferating tumors (Saitta et al. 2011). However, it is not possible to differentiate deoxyHb from other microscopic sources of susceptibility effects like tumor microbleeds. This might result in confounding results in glioblastomas, since microbleeds are a very typical characteristic of this type of brain tumor. It has turned out that the detection of microbleeds in high-grade gliomas is very helpful for discriminating them from other space-occupying pathologies such as demyelinating lesions, lymphomas, and metastases (see below).
Since microbleeds are best detected with high-resolution susceptibility-weighted imaging (SWI), this method plays an important role in tumor diagnosis. Since field inhomogeneities and thus susceptibility effects increase with the field strength, this relatively new MR technique benefits from higher field strengths which become increasingly available in the clinic. In SWI, filtered phase and magnitude information are combined to create a new susceptibility-weighted image contrast (Haacke et al. 2004). SWI visualizes normal or pathological venous structures and microbleeds that are not visible on conventional MR images. Pronounced intratumoral susceptibility signals (ITSS) are found in almost all glioblastomas but not in CNS lymphomas, demyelinating diseases, and low-grade gliomas (Mittal et al. 2009; Park et al. 2010; Peters et al. 2012). In most cases, microbleeds in metastases are less pronounced compared to microbleeds in glioblastomas (Park et al. 2010). Furthermore, intralesional venous structures differ considerably between different space-occupying brain lesions. The perivenous inflammation in multiple sclerosis (MS) lesions shows a typical SWI pattern revealing normal venous structures which are the lead structures for the MS plaques (Mittal et al. 2009). In contrast, high-grade gliomas not only show prominent microbleeds, but also a distorted and irregular neovasculature (Kim et al. 2009; Mittal et al. 2009).
4\ Longitudinal Relaxation Time T1
Several studies showed a positive correlation between the brain water content and the T1 relaxation time (MacDonald et al. 1986; Fatouros et al. 1991). The positive correlation of the mean diffusivity of water (Bastin 2002) and the T1 relaxation time and a significant T1 reduction under antiedematous therapy support the hypothesis that the T1 relaxation time reflects the tissue
water content (Andersen et al. 1993). Longer T1 values were found in enhancing lesions of MS patients compared to nonenhancing lesions, indicating a disruption of the BBB and hence a higher water content in the adjacent brain tissue prolonging T1 (Jurcoane et al. 2013). Human and animal studies showed prolonged T1 relaxation times in glioblastomas and their peritumoral edema compared to normal brain tissue (Englund et al. 1986; Hoehn-Berlage and Bockhorst 1994) and other tumors such as meningiomas and schwannomas. In contrast, necrotic tumor tissue might display a shortened T1 relaxation time which might be due to the presence of methemoglobin or mineralization (Bähr et al. 2011; Boyko et al. 1992). It should be mentioned that T1 shortening might also be a prognostic marker for the survival time of patients with recurrent glioblastomas under antiangiogenic therapy (Bähr et al. 2011, 2014).
The presence of contrast agents containing gadolinium (Gd) or manganese (Mn) shortens the T1 relaxation time considerably. Furthermore, co-registered maps of the longitudinal relaxation time before (qT1) and after application of a contrast agent (qT1+CA) allow for voxel-wise subtraction of (qT1+CA) from qT1 (Fig. 3). This approach enables the quantification of an even subtle contrast agent enhancement independent of visual detectability (Jurcoane et al. 2013), yielding sensitive quantification of the BBB damage (Jurcoane et al. 2013).
However, further studies are required to answer the question if T1 mapping, alone or in combination with T2 mapping, histograms of T1 and T2 relaxation times in the tumor tissue and edema, and more sophisticated three-dimensional tumor segmentation methods are valuable tools to delineate tumor tissue and edema and if these techniques are of help in differential diagnosis and therapy monitoring.
5\ Chemical Exchange Saturation
Transfer (CEST)
The CEST method is based on the magnetic transfer effects of specific protons in metabolites (and exogenous contrast agents) that are in chemical exchange with the solvent. The method for detecting CEST contrast is similar to the magnetization transfer (MT) contrast, i.e., the intensity change of the water signal is monitored in the presence of off-resonance saturation. A CEST effect is observed when the off-resonance frequency matches the MR frequency of a metabolite proton which is exchanging with the water protons at an appropriate rate. By varying the frequency, power, and timing of the saturation pulses, CEST contrast can be tuned to signals from endogenous mobile proteins and peptides (amide proton transfer, APT) (Zhou et al. 2003a, b) to amino protons of glutamate (gluCEST) (Cai et al. 2012) or to hydroxyl protons like those from glucose (glucoCEST) (Chan et al. 2012; Nasrallah et al. 2013). Since the basic principle of the contrast relies on proton exchange with the solvent and therefore depends on the concentration of solvent protons, the method allows to measure tissue pH (Zhou et al. 2003b).
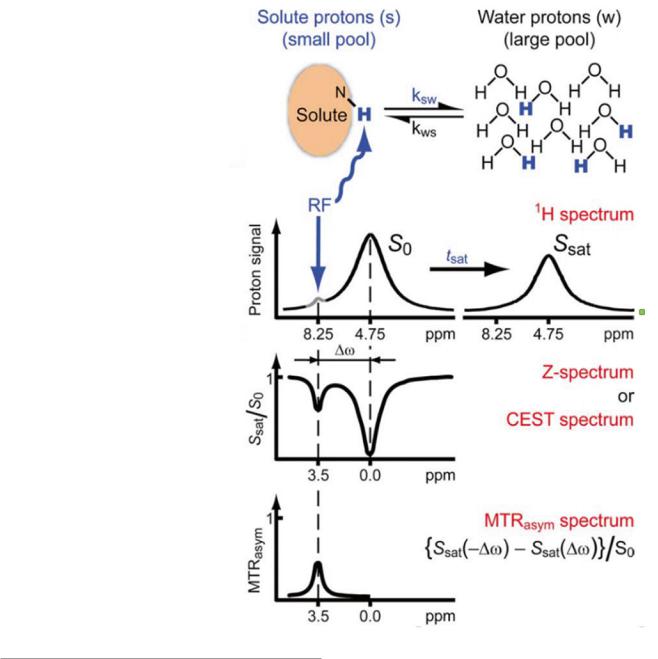
116 |
E. Hattingen et al. |
|
|
Fig. 4 Chemical exchange saturation transfer (CEST) method (With permission from van Zijl and Yadav (2011)). Further explanation is given in the text (Method)
a
b
c
d
6\ Cest Method
There are several articles which review the basic principles of CEST (Sherry and Woods 2008; van Zijl and Yadav 2011; van Zijl et al. 2003). Briefly, the method exploits the magnetization transfer (MT) effect which is well known from MT-MRI (Tofts 2003). In MTR contrast is generated by selectively irradiating off-resonance from the water signal, modifying the magnetization of macromolecules which are in contact with the water. By several mechanisms, this magnetization is transferred to the water protons detected with conventional MRI, causing a partial saturation and consequently a signal modification. The efficiency of the magnetization transfer is typically changed in several pathological
situations providing a surrogate marker for affected tissues (Tofts 2003). Like in MTR, CEST-MRI monitors the effect (signal reduction) of an off-resonance irradiation on the water signal; however, the target molecules for irradiation are rather small (amino acids, peptides, glucose, exogenous agents with specific properties) and have a well-defined typical resonance frequency which is known from MR spectroscopy (see Chap. MR Spectroscopic Imaging). Some of the protons in these molecules are exchanged with the solvent (water). Selective saturation of these protons will transfer their magnetization to the MRI-detectable water signal, enabling the quantification of the exchange partner by measuring the signal changes of the solvent.
The effect is shown in Fig. 4.

Advanced MR Methods in Differential Diagnosis of Brain Tumors |
117 |
|
|
The upper row (a) shows a scheme of the exchange mechanism, while the left part of row b depicts the MR spectrum of a sample with an aqueous solution containing a solute with amide protons (e.g., from polypeptides). On the left of the huge water signal at 4.75 ppm (S0), a weak signal at 8.25 ppm from exchangeable amide protons is visible. Saturation of these protons by RF irradiation at 8.25 ppm not only removes this signal but also causes a significant
decrease of the water signal (Ssat, right). The decrease of Ssat can be detected as contrast in MRI. Performing a series of
measurements with different offset frequencies for saturation provides a so-called Z-spectrum or CEST spectrum which is shown in row c. In the CEST spectrum, the normalized water signal (Ssat/So) in the presence of saturation is plotted versus the frequency difference between the water and the offset irradiation frequency ( ω). Obviously, irradiation at the water signal frequency will saturate the water protons causing a minimal Ssat (direct saturation, DS). In addition to DS, there is also the MT effect which reduces the water signal intensity in the presence of off-resonance irradiation. In a first approximation, DS and MT can be considered as independent of the sign of ω causing a symmetric Z-spectrum around the water frequency. In contrast, saturation of the water protons via exchange with saturated amide protons only appears when the saturation frequency matches the position of the amide protons (3.5 ppm to the left of water), resulting in an additional dip in Ssat/So at this frequency.
Defining the term MTRasym
\ |
{Ssat (− w) − Ssat ( w)} / S0 \ |
|
which determines the asymmetry of the MTR effect correct-
ing for DS and symmetric MT provides the MTRasym spectrum which is shown in row d. Chemical exchange is evident
as signal at the position of the exchanging amide protons. This signal reflects a change in water proton intensity and can be detected with methods known from MT-MRI. Compared to conventional MRSI, the methods have a huge potential for signal enhancement which increases with the exchange rate
kexchange but is counteracted by the T1 relaxation of tissue water. Also, the efficiency of saturation of solute protons by contin-
uous RF irradiation depends on the ratio ω/kexchange. Ideally ω/kexchange > > 1 (slow exchange regime) is required to allow sufficient saturation with reasonable RF power (van Zijl and
Yadav 2011), a condition which, at clinical field strength of 3 T, is only fulfilled for amide protons. Consequently, most applications on human brain tumors are focused on mobile proteins and polypeptides, measuring either their concentrations or tumor environmental changes which can modify the exchange rate (Zhou et al. 2003a; Togao et al. 2014). Amino and hydroxyl protons usually fall in the slow to medium
exchange regime ( ω/kexchange ~ 1), but it has been shown that glucose and glycogen can be detected with CEST in animal
models at high magnetic field strengths (>9 T, (Chan et al. 2012; Nasrallah et al. 2013; van Zijl et al. 2007)). Recently it was proposed that spin-locking (SL) techniques are more suited for the intermediate exchange regime (Lin et al. 2011; Jin et al. 2012) and pilot studies could detect myoinositol (Haris et al. 2011) and glutamate (Cai et al. 2012) using this method. However, no applications in oncology are published yet; thus, a description of these methods would be beyond the scope of this short overview.
In summary, for a metabolite with protons that are in exchange with the water, CEST provides a contrast which is specific to their concentration (like parameter images in MRSI) but with a signal intensity comparable to MT contrast. The following metabolites were detected via magnetization transfer mediated by chemical exchange of protons with the water:
•Amide protons in peptide bonds (endogenous mobile proteins and peptides) (APT-CEST)
•Glutamate (gluCEST)
•Glucose, glycogen, and myoinositol (glucoCEST)
Also, exogenous CEST agents have been introduced
(Ward et al. 2000), and their function as pH marker has been tested as proof of principle (Ward and Balaban 2000)
While the concept of CEST imaging is very intriguing, there are various pitfalls which might render the method as too complicated and up to now not very robust for routine clinical use:
•Like MT-MRS, the method requires a rather quantitative approach to correct for many competing mechanisms which can cause a decrease in signal intensity.
•Many parameters are affecting the exchange rate. Thus, the CEST-induced contrast might be ambiguous.
•Long measurement time and SAR problems.
7\ CEST Imaging in Brain Tumors
There is an increasing number of publications dealing with CEST in brain tumors. APT was introduced by Zhou et al. (2003b). First in vivo data from brain tumors were published by the same group 3 years later (Jones et al. 2006). A 3–4 % increased APT signal was found in brain tumors compared to peritumoral brain tissue or in animal models comparing glioma with radiation necrosis in rats (Zhou et al. 2008, 2011). Amide protons in the cytoplasm are thought to be the major source of the APT signal intensity. As known from FET-PET (Chap. Advanced MR Methods in Differential Diagnosis of Brain Tumors), glial brain tumors have an increased amino acid uptake compared to normal brain. Furthermore, an increased APT signal intensity was also found in necrotic high-grade gliomas, indicating that highly concentrated mobile proteins and peptides in the microcystic extracellular space might also increase APT signal intensity in tumors. In contrast to PET, the APT measures the endogenous content of amide protons without requiring external contrast agents.

118 |
E. Hattingen et al. |
|
|
Apart from the costs, this might be an advantage concerning repetitive follow-up examinations especially in young tumor patients. Recently, a study of thirty-six patients showed that APT signal intensity did not only differentiate between gliomas WHO grade II and IV, but also between gliomas WHO grade II and III and between gliomas WHO grade III and IV (Togaoetal.2014).Thus,APTseemstobeapromisingmethod for tumor grading and for depicting tumor heterogeneity. Referring to the experiences in FET-PET (Chap. Advanced MR Methods in Differential Diagnosis of Brain Tumors), APT might even be a promising method to differentiate between therapy-induced changes and tumor progression in brain tumor patients. As mentioned above, the exchange rate depends on the pH; thus, the tumor pH (Chap. MR Spectroscopic Imaging) also affects the APT signal intensity. On the other hand, pH can be measured with CEST, providing another parameter which could be exploited in tumor diagnosis. This requires to eliminate ambiguous influences between the pH and amide proton content, e.g., by combination with an independent pH measurement using phosphorus MR spectroscopy (Chap. MR Imaging of Brain Tumors).
Glycolysis, either anaerobic or aerobic, is enhanced in high-grade brain tumors, and an overexpression of glucose transporter (GLUT) proteins has been found in glioblastoma. An increased uptake of glucose in PET is a typical finding in malignant brain tumors. First results indicate that glucose concentrations can be detected by CEST via the hydroxyl protons (glucoCEST) (Nasrallah et al. 2013). Significant enhancement of the glucoCEST signal has been found upon systemic glucose infusion in an experimental study with breast cancer cells (Chan et al. 2012). However, to our knowledge, this method has not been evaluated for in vivo gliomas. It might be an interesting tool to evaluate alternative glioma therapies such as the low-carbohydrate, ketogenic diet (Rieger et al. 2014).
Although not yet applied in oncological systems, another variation of CEST with great potential is the depiction of chemical exchange saturation transfer effect between the amine group of glutamate and bulk water, also named gluCEST (Cai et al. 2012). Here, the CEST effect depends on the pH and glutamate concentration. Glutamate is released from glioma cells, possibly as by-product of GSH synthesis, an antioxidant which enhances the resistance to oxidative stress (Sontheimer 2008). In addition, glutamate is an important anaplerotic substrate which delivers α-ketoglutarate to the citrate cycle. It substitutes the intermediates of the citrate cycle which are used for protein biosynthesis in proliferating tumor cells. Glutamate can be transformed to glutamine through the glutamate-glutamine cycle of reactive astrocytes. Glutamine is a major metabolic fuel for both brain tumor cells and tumor-associated macrophages, which may create a microenvironment that facilitates aggressive growth of tumor cells. Using MR spectroscopy, increasing gluta-
mate concentrations were found in brain tumors (Kimura et al. 2007; Rijpkema et al. 2003). However, a reliable quantification of glutamate concentrations in tumor spectra is frequently hampered by the modulated baseline due to higher concentrations of lipids and macromolecules. Therefore, future investigations have to show whether gluCEST can provide reliable values for glutamate in tumors.
The methods based on chemical exchange contrast have a high potential to provide essential information on tumor biology, however, apart from CEST with amide protons (APT), these methods are still experimental. APT can be performed on clinical scanners and has been applied to human brain tumors providing promising results for diagnostic purposes. Since the CEST mechanism depends on metabolite concentration and pH, the reliability and robustness of the results have to be evaluated in studies with larger patient cohorts at different medical centers. In contrast, quantitative relaxometry and related methods are well suitable for standardized tumor diagnosis and monitoring, but their biological significance has to be validated in the future.
References
Andersen C, Haselgrove JC, Doenstrup S, Astrup J, Gyldensted C (1993) Resorption of peritumoural oedema in cerebral gliomas during dexamethasone treatment evaluated by NMR relaxation time imaging. Acta Neurochir (Wien) 122:218–224
Bähr O, Hattingen E, Rieger J, Steinbach JP (2011) Bevacizumab- induced tumor calcifications as a surrogate marker of outcome in patients with glioblastoma. Neuro Oncol 13:1020–1029
Bähr O, Harter PN, Weise LM, You SJ, Mittelbronn M, Ronellenfitsch MW, Rieger J, Steinbach JP, Hattingen E (2014) Sustained focal antitumor activity of bevacizumab in recurrent glioblastoma. Neurology 83:227–234
Bastin ME, Sinha S, Whittle IR, Wardlaw JM (2002) Measurements of water diffusion and T1 values in peritumoural oedematous brain. Neuroreport 13:1335–1340
Berghoff AS, Spanberger T, Ilhan-Mutlu A, Magerle M, Hutterer M, Woehrer A, Hackl M, Widhalm G, Christen T, Lemasson B, Pannetier N, Farion R, Remy C, Zaharchuk G, Barbier EL (2012) Is T2* enough to assess oxygenation? Quantitative blood oxygen level-dependent analysis in brain tumor. Radiology 262:495–502
Boyko OB, Burger PC, Shelburne JD, Ingram P (1992) Non-heme mechanisms for T1 shortening: pathologic, CT, and MR elucidation. AJNR Am J Neuroradiol 13:1439–1445
Cai K, Haris M, Singh A, Kogan F, Greenberg JH, Hariharan H, Detre JA, Reddy R (2012) Magnetic resonance imaging of glutamate. Nat Med 18:302–306
Chan KW, McMahon MT, Kato Y, Liu G, Bulte JW, Bhujwalla ZM, Artemov D, van Zijl PC (2012) Natural D-glucose as a biodegradable MRI contrast agent for detecting cancer. Magn Reson Med 68: 1764–1773
Cheng HL, Stikov N, Ghugre NR, Wright GA (2012) Practical medical applications of quantitative MR relaxometry. J Magn Reson Imaging 36:805–824
Damadian R (1971) Tumor detection by nuclear magnetic resonance. Science 171(3976):1151–1153
Englund E, Brun A, Larsson EM, Györffy-Wagner Z, Persson B (1986) Tumours of the central nervous system. Proton magnetic resonance
Advanced MR Methods in Differential Diagnosis of Brain Tumors |
119 |
|
|
relaxation times T1 and T2 and histopathologic correlates. Acta Radiol Diagn (Stockh) 27:653–659
Fatouros PP, Marmarou A, Kraft KA, Inao S, Schwarz FP (1991) In vivo brain water determination by T1 measurements: effect of total water content, hydration fraction, and field strength. Magn Reson Med 17:402–413
Haacke EM, Xu Y, Cheng YC, Reichenbach JR (2004) Susceptibility weighted imaging (SWI). Magn Reson Med 52:612–618
Harguindey S, Arranz JL, Wahl ML, Orive G, Reshkin SJ (2009) Proton transport inhibitors as potentially selective anticancer drugs. Anticancer Res 29:2127–2136. Review
Haris M, Cai K, Singh A, Hariharan H, Reddy R (2011) In vivo mapping of brain myo-inositol. Neuroimage 54:2079–2085
Hasan KM, Walimuni IS, Abid H, Datta S, Wolinsky JS, Narayana PA (2012) Human brain atlas-based multimodal MRI analysis of volumetry, diffusimetry, relaxometry and lesion distribution in multiple sclerosis patients and healthy adult controls: implications for understanding the pathogenesis of multiple sclerosis and consolidation of quantitative MRI results in MS. J Neurol Sci 313:99–109
Hattingen E, Jurcoane A, Daneshvar K, Pilatus U, Mittelbronn M, Steinbach JP, Bähr O (2013) Quantitative T2 mapping of recurrent glioblastoma under bevacizumab improves monitoring for non- enhancing tumor progression and predicts overall survival. Neuro Oncol 15:1395–1404
Hoehn-Berlage M, Bockhorst K (1994) Quantitative magnetic resonance imaging of rat brain tumors: in vivo NMR relaxometry for the discrimination of normal and pathological tissues. Technol Health Care 2:247–254
Hoehn-Berlage M, Tolxdorff T, Bockhorst K, Okada Y, Ernestus RI (1992) In vivo NMR T2 relaxation of experimental brain tumors in the cat: a multiparameter tissue characterization. Magn Reson Imaging 10:935–947
Hoskin PJ, Carnell DM, Taylor NJ, Smith RE, Stirling JJ, Daley FM, Saunders MI, Bentzen SM, Collins DJ, d’Arcy JA, Padhani AP (2007) Hypoxia in prostate cancer: correlation of BOLD-MRI with pimonidazole immunohistochemistry-initial observations. Int J Radiat Oncol Biol Phys 68:1065–1071
Jin T, Wang P, Zong X, Kim S-G (2012) Magnetic resonance imaging of the Amine–Proton EXchange (APEX) dependent contrast. Neuroimage 59:1218–1227
Jones CK, Schlosser MJ, van Zijl PC, Pomper MG, Golay X, Zhou J (2006) Amide proton transfer imaging of human brain tumors at 3 T. Magn Reson Med 56:585–592
Jurcoane A, Wagner M, Schmidt C, Mayer C, Gracien RM, Hirschmann M, Deichmann R, Volz S, Ziemann U, Hattingen E (2013) Within- lesion differences in quantitative MRI parameters predict contrast enhancement in multiple sclerosis. J Magn Reson Imaging 38:1454–1461
Kim HS, Jahng GH, Ryu CW, Kim SY (2009) Added value and diagnostic performance of intratumoral susceptibility signals in the differential diagnosis of solitary enhancing brain lesions: preliminary study. AJNR Am J Neuroradiol 30:1574–1579
Kimura T, Ohkubo M, Igarashi H, Kwee IL, Nakada T (2007) Increase in glutamate as a sensitive indicator of extracellular matrix integrity in peritumoral edema: a 3.0-tesla proton magnetic resonance spectroscopy study. J Neurosurg 106:609–613
Larocque MP, Syme A, Yahya A, Wachowicz K, Allalunis-Turner J, Fallone BG (2009) Temporal and dose dependence of T2 and ADC at 9.4 T in a mouse model following single fraction radiation therapy. Med Phys 36:2948–2954
Lin T, Autio J, Obata T, Kim SG (2011) Spin-locking versus chemical exchange saturation transfer MRI for investigating chemical exchange process between water and labile metabolite protons. Magn Reson Med 65:1448–1460
MacDonald HL, Bell BA, Smith MA, Kean DM, Tocher JL, Douglas RH, Miller JD, Best JJ (1986) Correlation of human NMR T1
values measured in vivo and brain water content. Br J Radiol 59:355–357
Mittal S, Wu Z, Neelavalli J, Haacke EM (2009) Susceptibility-weighted imaging: technical aspects and clinical applications, part 2. AJNR Am J Neuroradiol 30:232–252
Nasrallah FA, Pagès G, Kuchel PW, Golay X, Chuang KH (2013) Imaging brain deoxyglucose uptake and metabolism by glucoCEST MRI. J Cereb Blood Flow Metab 33:1270–1278
Oh J, Cha S, Aiken AH, Han ET, Crane JC, Stainsby JA, Wright GA, Dillon WP, Nelson SJ (2005) Quantitative apparent diffusion coefficients and T2 relaxation times in characterizing contrast enhancing brain tumors and regions of peritumoral edema. J Magn Reson Imaging 21:701–708
Park SM, Kim HS, Jahng GH, Ryu CW, Kim SY (2010) Combination of high-resolution susceptibility-weighted imaging and the apparent diffusion coefficient: added value to brain tumour imaging and clinical feasibility of non-contrast MRI at 3 T. Br J Radiol 83:466–475 Peters S, Knöß N, Wodarg F, Cnyrim C, Jansen O (2012) Glioblastomas versus lymphomas: More diagnostic certainty by using susceptibility-weighted imaging (SWI). Fortschr Roentgenstr 184:
713–718
Rieger J, Bähr O, Maurer GD, Hattingen E, Franz K, Brucker D, Walenta S, Kämmerer U, Coy JF, Weller M, Steinbach JP (2014) ERGO: a pilot study of ketogenic diet in recurrent glioblastoma. Int J Oncol 44:1843–1852
Rijpkema M, Schuuring J, van der Meulen Y, van der Graaf M, Bernsen H, Boerman R, van der KogelA, HeerschapA (2003) Characterization of oligodendrogliomas using short echo time 1H MR spectroscopic imaging. NMR Biomed 16:12–18
Saitta L, Heese O, Förster AF, Matschke J, Siemonsen S, Castellan L, Westphal M, Fiehler J, Goebell E (2011) Signal intensity in T2′ magnetic resonance imaging is related to brain glioma grade. Eur Radiol 21:1068–1076
Schad LR, Blüml S, Zuna I (1993) MR tissue characterization of intracranial tumors by means of texture analysis. Magn Reson Imaging 11:889–896
Sherry AD, Woods M (2008) Chemical exchange saturation transfer contrast agents for magnetic resonance imaging. Annu Rev Biomed Eng 10:391–411
Sontheimer H (2008) A role for glutamate in growth and invasion of primary brain tumors. J Neurochem 105:287–295
Tofts P (ed) Quantitative MRI of the brain: measuring changes caused by disease. John Wiley & Sons, Chichester, England in 2003. pp 272–298
Togao O, Yoshiura T, Keupp J, Hiwatashi A, Yamashita K, Kikuchi K, Suzuki Y, Suzuki SO, Iwaki T, Hata N, Mizoguchi M, Yoshimoto K, Sagiyama K, Takahashi M, Honda H (2014) Amide proton transfer imaging of adult diffuse gliomas: correlation with histopathological grades. Neuro Oncol 16:441–448
Tóth V, Förschler A, Hirsch NM, den Hollander J, Kooijman H, Gempt J, Ringel F, Schlegel J, Zimmer C, Preibisch C (2013) MR-based hypoxia measures in human glioma. J Neurooncol 115:197–207
van Zijl PC, Yadav NN (2011) Chemical exchange saturation transfer (CEST): what is in a name and what isn’t? Magn Reson Med 65:927–948
van Zijl PC, Zhou J, Mori N, Payen JF, Wilson D, Mori S (2003) Mechanism of magnetization transfer during on-resonance water saturation. A new approach to detect mobile proteins, peptides, and lipids. Magn Reson Med 49:440–449
van Zijl PC, Jones CK, Ren J, Malloy CR, Sherry AD (2007) MRI detection of glycogen in vivo by using chemical exchange saturation transfer imaging (glycoCEST). Proc Natl Acad Sci U S A 13(104):4359–4364
Ward KM, Balaban RS (2000) Determination of pH using water protons and chemical exchange dependent saturation transfer (CEST). Magn Reson Med 44:799–802
120 |
E. Hattingen et al. |
|
|
Ward KM, Aletras AH, Balaban RS (2000) A new class of contrast agents for MRI based on proton chemical exchange dependent saturation transfer (CEST). J Magn Reson 143:79–87
Zhou J, van Zijl PC (2006) Chemical exchange saturation transfer imaging and spectroscopy. Prog Nucl Magn Reson Spectrosc 48:109–136
Zhou J, Lal B, Wilson DA, Laterra J, van Zijl PC (2003a) Amide proton transfer (APT) contrast for imaging of brain tumors. Magn Reson Med 50:1120–1126
Zhou J, Payen JF, Wilson DA, Traystman RJ, van Zijl PC (2003b) Using the amide proton signals of intracellular proteins and peptides to detect pH effects in MRI. Nat Med 9:1085–1090
Zhou J, Blakeley JO, Hua J, Kim M, Laterra J, Pomper MG, van Zijl PC (2008) Practical data acquisition method for human brain tumor amide proton transfer (APT) imaging. Magn Reson Med 60:842–849
Zhou J, Tryggestad E, Wen Z, Lal B, Zhou T, Grossman R, Wang S, Yan K, Fu DX, Ford E, Tyler B, Blakeley J, Laterra J, van Zijl PC (2011) Differentiation between glioma and radiation necrosis using molecular magnetic resonance imaging of endogenous proteins and peptides. Nat Med 17:130–134