
- •Foreword
- •Preface
- •Contents
- •Symbols
- •1 Electromagnetic Field and Wave
- •1.1 The Physical Meaning of Maxwell’s Equations
- •1.1.1 Basic Source Variables
- •1.1.2 Basic Field Variables
- •1.1.3 Maxwell’s Equations in Free Space
- •1.1.4 Physical Meaning of Maxwell’s Equations
- •1.1.5 The Overall Physical Meaning of Maxwell’s Equations
- •1.2 Electromagnetic Power Flux
- •1.2.1 The Transmission of Electromagnetic Power Flux
- •1.2.2 Capacitors—Electrical Energy Storage
- •1.2.3 Inductor—Magnetic Energy Storage
- •1.2.4 Examples of Device Properties Analysis
- •1.3.1 Boundary Conditions of the Electromagnetic Field on the Ideal Conductor Surface
- •1.3.2 Air Electric Wall
- •2 Microwave Technology
- •2.1 The Theory of Microwave Transmission Line
- •2.1.1 Overview of Microwave Transmission Line
- •2.1.2 Transmission State and Cutoff State in the Microwave Transmission Line
- •2.1.3 The Concept of TEM Mode, TE Mode, and TM Mode in Microwave Transmission Line
- •2.1.4 Main Characteristics of the Coaxial Line [4]
- •2.1.5 Main Characteristics of the Waveguide Transmission Line
- •2.1.6 The Distributed Parameter Effect of Microwave Transmission Line
- •2.2 Application of Transmission Line Theories in EMC Research
- •3 Antenna Theory and Engineering
- •3.1 Field of Alternating Electric Dipole
- •3.1.1 Near Field
- •3.1.2 Far Field
- •3.2 Basic Antenna Concepts
- •3.2.1 Directivity Function and Pattern
- •3.2.2 Radiation Power
- •3.2.3 Radiation Resistance
- •3.2.4 Antenna Beamwidth and Gain
- •3.2.6 Antenna Feed System
- •4.1.1 Electromagnetic Interference
- •4.1.2 Electromagnetic Compatibility
- •4.1.3 Electromagnetic Vulnerability
- •4.1.4 Electromagnetic Environment
- •4.1.5 Electromagnetic Environment Effect
- •4.1.6 Electromagnetic Environment Adaptability
- •4.1.7 Spectrum Management
- •4.1.9 Spectrum Supportability
- •4.2 Essences of Quantitative EMC Design
- •4.2.2 Three Stages of EMC Technology Development
- •4.2.3 System-Level EMC
- •4.2.4 Characteristics of System-Level EMC
- •4.2.5 Interpretations of the EMI in Different Fields
- •4.3 Basic Concept of EMC Quantitative Design
- •4.3.1 Interference Correlation Relationship
- •4.3.2 Interference Correlation Matrix
- •4.3.3 System-Level EMC Requirements and Indicators
- •4.3.5 Equipment Isolation
- •4.3.6 Quantitative Allocation of Indicators
- •4.3.7 The Construction of EMC Behavioral Model
- •4.3.8 The Behavior Simulation of EMC
- •4.3.9 Quantitative Modeling Based on EMC Gray System Theory
- •5.2 Solution Method for EMC Condition
- •5.3 EMC Modeling Methodology
- •5.3.1 Methodology of System-Level Modeling
- •5.3.2 Methodology for Behavioral Modeling
- •5.3.3 EMC Modeling Method Based on Gray System Theory
- •5.4 EMC Simulation Method
- •6.1 EMC Geometric Modeling Method for Aircraft Platform
- •6.2.1 Interference Pair Determination and Interference Calculation
- •6.2.2 Field–Circuit Collaborative Evaluation Technique
- •6.2.3 The Method of EMC Coordination Evaluation
- •6.3 Method for System-Level EMC Quantitative Design
- •6.3.2 The Optimization Method of Single EMC Indicator
- •6.3.3 The Collaborative Optimization Method for Multiple EMC Indicators
- •7.1 The Basis for EMC Evaluation
- •7.2 The Scope of EMC Evaluation
- •7.2.1 EMC Design
- •7.2.2 EMC Management
- •7.2.3 EMC Test
- •7.3 Evaluation Method
- •7.3.1 The Hierarchical Evaluation Method
- •7.3.2 Evaluation Method by Phase
- •8 EMC Engineering Case Analysis
- •8.1 Hazard of Failure in CE102, RE102, and RS103 Test Items
- •8.2 The Main Reasons for CE102, RE102, and RS103 Test Failures
- •8.2.1 CE102 Test
- •8.2.2 RE102 Test
- •8.2.3 RS103 Test
- •8.3 The Solutions to Pass CE102, RE102, and RS103 Tests
- •8.3.1 The EMC Failure Location
- •8.3.2 Trouble Shooting Suggestions
- •A.1 Pre-processing Function
- •A.2 Post-processing Function
- •A.3 Program Management
- •A.4 EMC Evaluation
- •A.5 System-Level EMC Design
- •A.6 Database Management
- •References

2.1 The Theory of Microwave Transmission Line |
31 |
2.1.4 Main Characteristics of the Coaxial Line [4]
The working mode of the coaxial line is TEM wave. In the traveling wave state (electromagnetic wave propagates forward in coaxial line), the average power that can pass through the coaxial line can be expressed as
P Zc I 2 |
2 |
(2.10) |
In order to avoid the breakdown in the coaxial line, it is required that the maximum power allowed in the traveling wave state in the coaxial line should meet
|
b2 |
2 |
D |
|
|
Pmax |
|
Eb ln |
|
|
(2.11) |
120 |
|
d |
where Eb is the magnitude of the electric field intensity at the inner conductor of the coaxial line at the time of breakdown, b is the radius of the inner conductor, d is the diameter of the inner conductor, and D is the diameter of the outer conductor.
If there is loss from the inner and outer conductors of the coaxial line, the surface resistance of the inner conductor is Rsd , and the surface resistance of the outer conductor is Rs D , then the attenuation coefficient of the coaxial line can be expressed as
α |
1 |
Rs D |
|
Rsd |
|
|
|
|
|
+ |
|
(2.12) |
|
120 π ln Dd |
|
D |
d |
If the medium is filled between the inner and outer conductors of the coaxial line, the loss of the medium should also be considered. The best working state of the coaxial line is when filled with nondispersion medium and working in TEM wave mode (dispersion medium is a medium in which the propagation speed of electromagnetic waves is a function of frequency. Any medium with loss is dispersion medium.). However, when the operating frequency of the electromagnetic wave is sufficiently high, a high-order mode occurs in the coaxial line. Therefore, in order to ensure that the coaxial line is in a single-mode transmission state, there are certain restrictions toward the inner and outer diameter dimensions and operating frequency of the coaxial line.
The inner and outer dimensions of the coaxial line should meet the requirement
D
< 4 and λ > 1.73(D + d)
d
Based on the calculation, the size of the coaxial line can be considered from two aspects.
(1)The maximum passing power: In the case of fixed diameter of the outer conductor of the coaxial line, the maximum corresponding power capacity of the coaxial
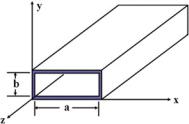
32 |
2 Microwave Technology |
line is: The inner–outer radius ratio is 1.65, and the characteristic impedance is
30.
(2)The minimum attenuation coefficient: In the case of fixed diameter of the outer conductor of the coaxial line, the minimum attenuation condition of the coaxial line is: The inner–outer radius ratio is 3.6, and the characteristic impedance is
77.
At present, the coaxial line with 75 or 50 is usually used according to past experiences, the former has minimum attenuation, and the latter has large passing power and small attenuation coefficient.
2.1.5Main Characteristics of the Waveguide Transmission Line
The most common waveguide transmission lines are rectangular waveguides and circular waveguides. The boundary conditions of the two are different due to the different geometrical structures. Here, the main characteristics of the waveguide transmission line will be introduced from both a general and the EMC design perspective.
There is no TEM wave in a rectangular waveguide, but there can be TE waves and TM waves. The characteristics of the TE wave and the TM wave in the rectangular waveguide are analyzed below.
As mentioned in Sect. 2.1.2, kc is an important parameter, and it gives the propagation conditions of different modes.
As shown in Fig. 2.5, in a rectangular waveguide, according to the size of the waveguide (a is the wide side, and b is the narrow side), kc can be expressed as
2 |
|
mπ 2 |
|
nπ 2 |
, (m, n 0, 1, 2, . . .) |
|
||||
(kc)mn |
|
|
|
+ |
|
|
|
(2.13) |
||
|
a |
|
b |
where m and n represent the various modes that can exist in the waveguide. m and n can be any positive integer or zero (they cannot be zero at the same time; otherwise, a nonzero solution cannot be obtained).
Fig. 2.5 Rectangular waveguide transmission lines
2.1 The Theory of Microwave Transmission Line |
33 |
Different m and n correspond to different TE waves and TM waves, denoted as T Emn and T Mmn , or we can use H mn instead of T Emn and Emn instead of T Mmn . T Emn can be explained as: T means transverse, so TE refers to transverse electric wave. H mn can be explained as: There is a magnetic field (H field) in the direction of propagation. Therefore, the two are only expressed differently.
T Emn and T Mmn are also known as the mode (waveform) in the waveguide transmission line, and m and n represent the mode number. For different modes, there are different cutoff wavelengths:
(λc)mn |
2π |
|
|
|
|
2 |
|
|
|
, (m, n 0, 1, 2, . . .) |
(2.14) |
|
(kc)mn |
|
|
|
|
|
|
|
|
||||
m |
|
2 |
+ |
n |
|
2 |
||||||
|
|
|
a |
|
b |
|
|
|
Only the modes that meet the propagation requirement λ < (λc)mn (refer to the transmission state and cutoff state in microwave transmission lines in Sect. 2.1.2) can be propagated in a rectangular waveguide.
For any given a, b, the larger m and n are, the larger the corresponding cutoff frequency fc is. In all possible modes, the cutoff wavelength λc corresponding to the T E10 mode is the maximum, and the corresponding cutoff frequency fc is the minimum. Therefore, T E10 is called the lowest mode in a rectangular waveguide (or a fundamental mode), and other modes are called higher-order modes.
When T E10 is in the traveling wave state, the theoretical value of the maximum power that can pass is
|
ab |
2 |
|
|
λ |
|
2 |
|
|
|
|
|
|||||
Pmax |
|
Eb |
|
1 − |
|
|
(2.15) |
|
480π |
2a |
|
Considering some practical factors results in the drop of the maximum power, and the maximum power allowed by waveguide in engineering is 25–30% of the theoretical value.
The attenuation coefficient of T E10 is
α |
|
Rs |
|
|
|
1 + 2 × |
b |
λ |
2 |
|||
|
|
|
|
|
|
|
|
|
|
(2.16) |
||
|
|
|
|
|
|
|
a |
2a |
||||
120π b |
1 − |
λ |
|
2 |
|
|||||||
|
|
2a |
|
|
|
|
|
|
|
Obviously, the larger the cross-sectional size of the waveguide, the smaller the attenuation. However, an increase in the cross-sectional size leads to a multi-mode phenomenon. Therefore, the size of the rectangular waveguide needs to be balanced.
For the rectangular waveguide with a single transmission mode T E10, the size selection principles are: (1) Single-mode transmission should be guaranteed; (2) loss and attenuation should be as small as possible; (3) the power capacity should be as large as possible; (4) the dispersion should be as small as possible.
34 |
2 Microwave Technology |
The waveguide of which b a/2 can be taken as the standard waveguide form, which has the characteristics of the maximum passing power with a guaranteed bandwidth.
It is worth pointing out that the above explanations emphasize T E10 in the traveling wave state. An important condition for ensuring the traveling wave state is that the electromagnetic wave is far from the electromagnetic discontinuity; that is, the cross-sectional dimension of the waveguide is much smaller than the longitudinal length.
When the longitudinal length is not very long, the high-order mode will not be completely in the off state, which is called the withering mode (having a certain propagation ability, but the amplitude is gradually attenuated). The degree of attenuation of the withering mode is related to the longitudinal length. The longer the longitudinal length, the greater the attenuation of the withering mode. It is a very important concept in the structural design of shielding.
The field solution of a circular waveguide is different from that of a rectangular waveguide, but its basic principle and analysis method are similar. Circular waveguides also have single-mode transmission problems, high-order mode problems, cutoff wavelengths, etc. We will not discuss the details here. Our readers can refer to reference [3] for more explanation.
2.1.6The Distributed Parameter Effect of Microwave Transmission Line
The distributed parameter effect is produced when the length of the microwave transmission line is much longer than that of the electromagnetic wave. It is also called the long-term theory in some contexts. It is important to note that the length of the transmission line does not depend on its geometric length, but depends on its electric length, which is defined as the ratio of geometric length to wavelength. The distributed parameter effect is called “electric length” long when the phase of the electric length is not equal everywhere. For example, a 50 km transmission line is not considered to be very long because it operates at 50 Hz and has a wavelength of 6,000 km. But a 10 cm transmission line that works at 10 GHz is considered to be very long, because the wavelength of 10 GHz is only 3 cm.
This book will use the transmission line theory to analyze the distributed parameter effect problem. Figure 2.6 shows a uniform transmission line and circuit model. In this figure, a finite length is cut off on the coming line (upper line) and the returning line (lower line) and is divided into several differential segments, each of which has its own unit length resistance, self-inductance, and mutual inductance.
Taking two sets of independent equations in integral form which are derived from Maxwell’s equations as basic laws, the equation of uniform transmission line with mutual inductance can be derived [6]
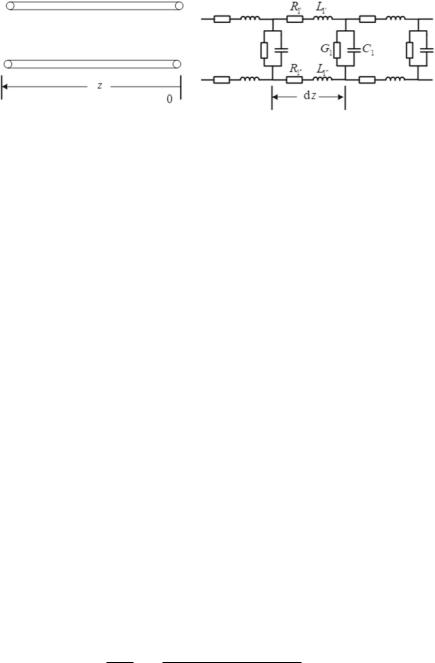
2.1 The Theory of Microwave Transmission Line |
35 |
(a) |
(b) |
|
|
Energy |
Load |
Fig. 2.6 Transmission lines and their equivalent circuits. a Transmission lines and b equivalent circuit
− |
dV dz |
|
(R1 |
|
+ R1 |
|
)I + jω(L1 |
|
+ L1 |
|
+ M1 |
|
+ M1 |
)I |
˙ |
|
|
˙ |
|
|
|
˙ |
|||||||
− |
˙ |
|
˙ |
|
|
˙ |
|
|
|
|
|
|
(2.17) |
|
|
d I dz |
|
G1 V + jωC1 V |
|
|
|
|
|
|
where R1 , R1 , L1 , L1 , M1 , and M1 , respectively, represent the resistances, selfinductance coefficients, and mutual inductance coefficients of unit length of the incoming line and the return line.
When the mutual inductance of the transmission line is ignored, and there is R1 R1 + R1 and L1 L1 + L1 , then Eq. (2.17) can be simplified as:
− |
˙ |
dz |
|
Z |
˙ |
|
|
dV |
|
1 I |
|
||
− |
˙ |
|
|
|
˙ |
(2.18) |
|
d I dz |
|
Y1 V |
where Z1 is the series impedance of unit length, Z1 R1 + jωL1; Y1 is the parallel admittance of unit length, Y1 G1 + jωC1.
The equation of voltage and current on the line is also called the telegraph equation. The above equations show that the voltage change on the transmission line is caused by the reduction of the series impedance. The change in current is caused by the shunt action of the shunt admittance.
By integrating the telegraph Eq. (2.18), we can get
|
|
|
˙ |
dz2 |
|
|
˙ |
|
˙ |
|
(2.19) |
|
|
|
d2 V |
|
Z1Y1 V |
γ 2 V |
|
||||
The general solution is |
|
|
|
|
|
|
|
|
|
||
|
˙ |
˙ |
˙ |
|
|
˙ |
˙ |
|
− |
˙ |
(2.20) |
|
V |
V1eγ z + V2e−γ z and I |
V1eγ z |
|
V2e−γ z Zc |
||||||
V |
and V are the undetermined complex constant, γ is the propagation con- |
||||||||||
where ˙1 |
˙2 |
|
|
|
|
|
|
|
|
|
|
stant, and Zc is the characteristic impedance. The formula of γ and Zc is
γ Z1Y1 ( R1 + jωL1)(G1 + jωC1)

36 |
2 Microwave Technology |
Zc Z1 γ Z1 Y1 ( R1 + jωL1) (G1 + jωC1)
The voltage and current of the incident wave are:
˙ |
|
˙ |
˙ |
|
˙ |
V+ |
|
V1eγ z , |
I+ |
|
V1eγ z Zc |
The voltage and current of the reflected wave are:
˙− ˙ |
˙− − ˙ |
||
V |
V2e−γ z , |
I |
V2e−γ z Zc |
(2.21)
(2.22a)
(2.22b)
The relationship between the voltage and current of the incident wave and characteristic impedance, and the relationship between voltage and current of the reflected wave and characteristic impedance are as follows
|
˙ |
˙ |
− ˙− |
˙− |
(2.23) |
Zc |
V+ |
I+ |
V |
I |
However, the ratio of the total voltage to the total current in a transmission line in general is not equal to the characteristic impedance.
The total voltage in the transmission line is
˙ |
|
˙+ |
˙− |
(2.24a) |
V |
|
V |
+ V |
The total current in the transmission line is
˙ ˙ |
˙− |
˙ |
− ˙− |
|
|
I I+ + I |
V+ |
V |
Zc |
(2.24b) |
If the loss of the microwave transmission line is assumed to be small enough, we have R1 << ωL1, G1 << ωC1. When the loss of the transmission line can be ignored, the characteristic parameters of the nondestructive transmission line can be obtained.
The propagation constant is
γ jω |
L1C1 |
jβ |
(2.25) |
|||||
The phase constant is |
|
|
|
|
|
|||
β ω |
|
|
|
(2.26) |
||||
L1C1 |
||||||||
The characteristic impedance is |
|
|
|
|
|
|||
|
|
|
|
|
|
|
|
|
|
|
|
|
|
|
|
||
Zc L1 |
C1 |
(2.27) |
2.1 The Theory of Microwave Transmission Line |
37 |
The general solution of the nondestructive transmission line is:
˙ |
|
˙ |
˙ |
˙ |
˙ |
− |
˙ |
(2.28) |
|
V |
|
V1e jβ z + V2e− jβ z , |
I |
V1e jβ z |
|
V2e− jβ z Zc |
|||
|
|
|
V |
is ϕ |
, and the initial phase angle of V is ϕ |
; |
|||
Assume the initial phase angle of ˙1 |
1 |
|
|
˙2 |
2 |
|
the relationship between voltage and current in Fig. 2.6 can be obtained. Since the
|
V |
(V ) |
z-axis in Fig. 2.6 is from the load to the source, the incoming voltage wave ˙+ |
˙1 |
|
jβ z |
V (V ) propagates |
|
propagates in –z-direction with the factor e ; the reflection wave ˙− ˙2 |
|
in +z-direction with the factor e−jβ z (let the time factor be ejωt ).
1. Reflection coefficient
The reflection coefficient is defined as the ratio of the reflected voltage to the incident voltage, that is
|
| |
|
| |
ejθ |
|
˙− |
˙ |
|
V2ej(ϕ2 |
−ϕ1−2β z) V1 |
(2.29) |
Γ |
|
Γ |
|
|
V |
V+ |
|
The reflection coefficient is a function of the reference position. If the reference position is set at z 0, the reflection coefficient of the terminal load is
|
|
| |
|
|
| |
ejθ0 |
|
˙− |
˙ |
|
V2ej(ϕ2 |
−ϕ1) V1 |
(2.30) |
||
Γ0 |
|
|
Γ0 |
|
|
V |
V+ |
|
|||||||
where |
|
|
|
|
|
|
|
|
|
|
|
|
|
|
|
|
| |
Γ0 |
| |
|
V2 V1, θ0 |
|
(ϕ2 |
− |
ϕ1) |
|
|||||
|
|
|
|
|
|
|
|
There is the following relationship between the reflection coefficient Γ at any position z and the reflection coefficient Γ0 at the reference point
Γ Γ0ej−2β z |Γ |ejθ .
Apparently, there is
|Γ | |Γ0|, θ θ0 − 2β z |
(2.31) |
Equation (2.31) provides the transformation formula of the reflection coefficient when the reference surface moves: When the reference surface moves, the modulus of the reflection coefficient on the nondestructive transmission line remains unchanged, and the phase is linearly related to the moving distance.
2. Input impedance
The ratio of the total voltage to the total current on the reference surface is defined as the input impedance, and its reciprocal is the input admittance.
|
|
1 Yi |
˙ |
˙ |
(2.32) |
Zi |
|
V |
I |
38 |
|
|
|
|
|
|
|
|
|
|
|
|
|
|
|
|
|
|
|
|
|
|
|
|
|
|
|
2 |
Microwave Technology |
|
Input impedance can also be expressed with reflection coefficient |
|
|||||||||||||||||||||||||||||
|
|
|
1 |
|
|
|
|
˙ |
|
|
|
˙+ |
|
|
˙− |
|
|
|
|
1 + Γ |
|
|
||||||||
|
|
Zi |
|
|
|
|
|
V |
|
Zc |
V |
|
+ V |
|
Zc |
|
|
(2.33) |
||||||||||||
|
|
Y |
i |
|
|
I |
|
V |
|
|
|
V |
1 |
− |
Γ |
|
||||||||||||||
|
|
|
|
|
|
|
|
˙ |
|
|
|
˙+ − ˙− |
|
|
|
|
|
|
|
|
|
|||||||||
and then we have |
|
|
|
|
|
|
|
|
|
|
|
|
|
|
|
|
|
|
|
|
|
|
|
|
|
|
|
|
||
|
|
|
|
|
|
|
Γ |
|
|
Zi − Zc |
|
|
|
Yc − Yi |
|
|
|
|
|
|
|
(2.34) |
||||||||
|
|
|
|
|
|
|
|
Zi + Zc |
Yc + Yi |
|
|
|
|
|
|
|||||||||||||||
|
|
|
|
|
|
|
|
|
|
|
|
|
|
|
|
|
||||||||||||||
3. Terminal equation |
|
|
|
|
|
|
|
|
|
|
|
|
|
|
|
|
|
|
|
|
|
|
|
|
|
|
|
|
||
Using the terminal condition at z |
|
0, constants V |
|
|
|
V can be determined. |
||||||||||||||||||||||||
Since z 0, we can get: |
|
|
|
|
|
|
|
|
|
|
|
˙1 and |
|
˙2 |
|
|
|
|||||||||||||
|
|
|
|
|
|
|
|
|
|
|
|
|
|
|
|
|
|
|
|
|
|
|
|
|
|
|||||
|
|
˙ |
|
|
|
˙ |
|
|
|
|
|
˙ |
|
|
|
|
z 0 |
|
|
˙ |
|
|
|
˙ |
|
|
||||
|
˙ |
V0 |
|
|
V1ejβ z + V2e−jβ z |
|
V1 |
|
+ V2 |
|
(2.35a) |
|||||||||||||||||||
|
˙ |
|
|
|
|
− |
|
˙ |
|
|
|
|
|
|
|
z 0 |
|
˙ |
|
|
− |
˙ |
|
|
||||||
|
I0 |
V1ejβ z |
|
V2e−jβ z Zc |
|
V1 |
|
|
V2 |
Zc |
(2.35b) |
|||||||||||||||||||
V |
and I |
are the voltage and current at z |
|
0. |
|
|
|
|
|
|
|
|||||||||||||||||||
where ˙0 |
˙0 |
|
|
|
|
|
|
|
|
|
|
|
|
|
|
|
|
|
|
|
|
|
|
|
|
|
|
|
|
|
Then, |
|
|
|
|
|
|
|
|
|
|
|
|
|
|
|
|
|
|
|
|
|
|
|
|
|
|
|
|
|
|
|
|
|
|
|
|
|
|
˙ |
|
|
˙ |
|
|
˙ |
|
|
|
|
|
|
|
|
|
|
|
|
|
|
||
|
|
|
|
|
|
|
V1 |
|
V0 |
+ I0 Zc 2 |
|
|
|
|
|
|
|
|
|
|||||||||||
|
|
|
|
|
|
|
|
˙ |
|
˙ |
− |
˙ |
|
|
|
2 |
|
|
|
|
|
|
|
|
(2.36) |
|||||
|
|
|
|
|
|
|
V2 |
|
|
V0 |
|
|
I0 Zc |
|
|
|
|
|
|
|
|
The solution to the terminal equation can be obtained by putting the terminal Eq. (2.36) into the terminal Eqs. (2.35a, 2.35b)
˙ |
˙0 |
|
˙0 |
Z |
c |
sin β z |
|
|||
V |
V |
cos β z + jI |
|
|
|
|||||
|
|
|
˙0 |
|
|
|
|
|||
I˙ I˙0 cos β z + j |
V |
|
|
|
sin β z |
(2.37) |
||||
Zc |
|
|||||||||
The terminal Eq. (2.37) relates the voltage |
V and the current I on the reference |
|||||||||
|
˙ |
|
|
|
˙ |
|||||
surface from the terminal load z to the terminal load voltage |
V and terminal load |
|||||||||
˙0 |
||||||||||
current I˙0. |
|
|
|
|
|
|
|
|
|
|
Using the terminal Eq. (2.37), the relationship between the input impedance Zi and the input admittance Yi , with the terminal load impedance Z0 and the terminal load admittance Y0, can be obtained as
|
|
|
V |
|
|
|
Z |
0 |
cos β z + jZ |
c |
sin β z |
|
|
Z |
0 |
+ jZ |
c |
tg β z |
|
||||||
|
Zi |
˙ |
Zc |
|
|
|
|
|
Zc |
|
|
|
|
|
|
||||||||||
|
I˙ |
|
Zc cos β z + jZ0 sin β z |
Zc + jZ0tg β z |
|
||||||||||||||||||||
|
Yi |
|
I˙ |
|
Yc |
Y0 cos β z + jYc sin β z |
|
Yc |
Y0 + jYctg β z |
|
(2.38) |
||||||||||||||
|
V |
Y cos β z + jY |
0 |
sin β z |
Y + jY |
tg β z |
|||||||||||||||||||
|
|
|
˙ |
|
|
|
c |
|
|
|
|
|
|
|
c |
|
0 |
|
|
|
|
|
|||
where Z0 |
|
1 Y0 |
|
˙ |
|
|
|
˙ |
|
|
|
|
|
|
|
|
|
|
|
|
|
|
|
||
|
|
V0 |
I0. |
|
|
|
|
|
|
|
|
|
|
|
|
|
|
2.1 The Theory of Microwave Transmission Line |
39 |
4. The state of traveling wave, pure standing wave, and traveling–standing wave in transmission line
Because the load in the transmission line is different, the state in the transmission line is also different. Generally, there are three typical states: traveling wave state, pure standing wave state, and traveling–standing wave state.
(1) Traveling wave state
If the load absorbs all the incident energy and there is no reflection, it is only a one-way transmission from the source to the load in the transmission line. This state
is called a traveling wave state. |
|
|
|
|
Because the reflected wave is zero, i.e., |
V |
|
0, we can get |
|
˙− |
|
|||
V |
V |
|
jβ z |
(2.39) |
˙ |
˙1e |
|
||
˙ |
˙ |
|
|
(2.40) |
I |
V ejβ z Zc |
Both sides of Eqs. (2.39) and (2.40) are multiplied with ejωt , and the instantaneous
voltage and current expression can be obtained |
|
||
v(z, t) V1 sin(ωt − β z + ϕ1) |
(2.41a) |
||
i(z, t) |
V1 |
|
|
|
sin(ωt − β z + ϕ1) |
(2.41b) |
|
Zc |
(2) Pure standing wave state
If the active power is not absorbed by the load, the power of the incident wave will be reflected completely. This state is called the pure standing wave state. When the load is fully reflected, the modulus of the reflection coefficient must be 1, i.e.,
| |
Γ0 |
| |
− |
Zc) |
|
|
1 |
|
(Z0 |
|
(Z0 + Zc) |
|
|Z0 − Zc| |Z0 + Zc|
Zc of the transmission line without consumption is a real number, and the above formula is only valid when Z0 0, Z0 ∞, or Z0 jX0, that is, in the situation of short circuit, open circuit, or pure reactance load, respectively.
The voltage and current on the transmission line are
˙ |
|
˙ |
|
|
˙ |
|
(2.42a) |
V |
|
jI0 Zc sin β z |
|
jI0 Zc sin 2πz λ |
|||
|
˙ |
˙ |
|
˙ |
|
|
|
|
I |
|
I0 cos β z |
|
I0 cos 2πz |
λ |
(2.42b) |
The instantaneous value is
40 |
2 |
Microwave Technology |
|
v(z, t) I0 Zc sin 2πz λ cos(ωt + ϕ0) |
(2.43a) |
|
i(z, t) I0 cos 2πz λ sin(ωt + ϕ0) |
(2.43b) |
By comparing the pure standing wave with the traveling wave, we see the following differences:
(1)In the pure standing wave state, the voltage and the current along the line no longer have the characteristics of forward propagation, but they simply oscillate in place; in λ/2, the magnitude of each point is different, but the phase is exactly the same; but in the other λ/2, the phase is completely opposite.
(2)There is a 90° phase difference between the voltage and the current of the pure standing wave, and the power in this situation is reactive power.
(3)There is a 90° phase difference between the voltage and current in space.
(3) Traveling–standing wave state
If the load absorbs some of the energy of the incident wave, the rest is reflected back. This state between the traveling wave and the pure standing wave is called the traveling–standing wave state. The load corresponding to the standing wave state is neither characteristic impedance nor open circuit, short circuit, or pure reactance.
The expression of traveling–standing wave voltage is
˙ |
|
˙ |
˙ |
|
˙ |
˙ |
− |
˙ |
ejβ z , |
˙ |
|
˙ |
|
V |
|
V1ejβ z + V2e−jβ z |
|
2V2 cos β z + |
V1 |
|
V2 |
( V1 |
|
> V2 |
) (2.44) |
where the first term is pure standing wave and the second term is traveling wave. It can be seen from Eq. (2.44) that the traveling–standing wave is actually the
superposition of the traveling wave and the pure standing wave.
5. Voltage standing wave ratio
The voltage standing wave ratio ρ is the ratio of the maximum voltage to the minimum voltage, i.e.,
ρ Vmax Vmin |
(2.45) |
The maximum voltage occurs when the incident wave voltage is in phase with the reflected wave voltage, i.e., Vmax V+ + V−. The voltage minimum occurs when the incident wave voltage and the reflected wave voltage are opposite, i.e., Vmin V+ − V−. So, the voltage standing wave ratio can be expressed as
ρ |
|
V+ + V− |
|
1 + |Γ | |
(2.46) |
|
V+ − V− |
1 − |Γ | |
|||||
|
|
After proper transformation of (2.46), we have
Γ |
|
ρ − 1 |
(2.47) |
|
ρ + 1 |
||||
|
|