
- •Table of Contents
- •Copyright
- •Dedication
- •Introduction to the eighth edition
- •Online contents
- •List of Illustrations
- •List of Tables
- •1. Pulmonary anatomy and physiology: The basics
- •Anatomy
- •Physiology
- •Abnormalities in gas exchange
- •Suggested readings
- •2. Presentation of the patient with pulmonary disease
- •Dyspnea
- •Cough
- •Hemoptysis
- •Chest pain
- •Suggested readings
- •3. Evaluation of the patient with pulmonary disease
- •Evaluation on a macroscopic level
- •Evaluation on a microscopic level
- •Assessment on a functional level
- •Suggested readings
- •4. Anatomic and physiologic aspects of airways
- •Structure
- •Function
- •Suggested readings
- •5. Asthma
- •Etiology and pathogenesis
- •Pathology
- •Pathophysiology
- •Clinical features
- •Diagnostic approach
- •Treatment
- •Suggested readings
- •6. Chronic obstructive pulmonary disease
- •Etiology and pathogenesis
- •Pathology
- •Pathophysiology
- •Clinical features
- •Diagnostic approach and assessment
- •Treatment
- •Suggested readings
- •7. Miscellaneous airway diseases
- •Bronchiectasis
- •Cystic fibrosis
- •Upper airway disease
- •Suggested readings
- •8. Anatomic and physiologic aspects of the pulmonary parenchyma
- •Anatomy
- •Physiology
- •Suggested readings
- •9. Overview of diffuse parenchymal lung diseases
- •Pathology
- •Pathogenesis
- •Pathophysiology
- •Clinical features
- •Diagnostic approach
- •Suggested readings
- •10. Diffuse parenchymal lung diseases associated with known etiologic agents
- •Diseases caused by inhaled inorganic dusts
- •Hypersensitivity pneumonitis
- •Drug-induced parenchymal lung disease
- •Radiation-induced lung disease
- •Suggested readings
- •11. Diffuse parenchymal lung diseases of unknown etiology
- •Idiopathic pulmonary fibrosis
- •Other idiopathic interstitial pneumonias
- •Pulmonary parenchymal involvement complicating systemic rheumatic disease
- •Sarcoidosis
- •Miscellaneous disorders involving the pulmonary parenchyma
- •Suggested readings
- •12. Anatomic and physiologic aspects of the pulmonary vasculature
- •Anatomy
- •Physiology
- •Suggested readings
- •13. Pulmonary embolism
- •Etiology and pathogenesis
- •Pathology
- •Pathophysiology
- •Clinical features
- •Diagnostic evaluation
- •Treatment
- •Suggested readings
- •14. Pulmonary hypertension
- •Pathogenesis
- •Pathology
- •Pathophysiology
- •Clinical features
- •Diagnostic features
- •Specific disorders associated with pulmonary hypertension
- •Suggested readings
- •15. Pleural disease
- •Anatomy
- •Physiology
- •Pleural effusion
- •Pneumothorax
- •Malignant mesothelioma
- •Suggested readings
- •16. Mediastinal disease
- •Anatomic features
- •Mediastinal masses
- •Pneumomediastinum
- •Suggested readings
- •17. Anatomic and physiologic aspects of neural, muscular, and chest wall interactions with the lungs
- •Respiratory control
- •Respiratory muscles
- •Suggested readings
- •18. Disorders of ventilatory control
- •Primary neurologic disease
- •Cheyne-stokes breathing
- •Control abnormalities secondary to lung disease
- •Sleep apnea syndrome
- •Suggested readings
- •19. Disorders of the respiratory pump
- •Neuromuscular disease affecting the muscles of respiration
- •Diaphragmatic disease
- •Disorders affecting the chest wall
- •Suggested readings
- •20. Lung cancer: Etiologic and pathologic aspects
- •Etiology and pathogenesis
- •Pathology
- •Suggested readings
- •21. Lung cancer: Clinical aspects
- •Clinical features
- •Diagnostic approach
- •Principles of therapy
- •Bronchial carcinoid tumors
- •Solitary pulmonary nodule
- •Suggested readings
- •22. Lung defense mechanisms
- •Physical or anatomic factors
- •Antimicrobial peptides
- •Phagocytic and inflammatory cells
- •Adaptive immune responses
- •Failure of respiratory defense mechanisms
- •Augmentation of respiratory defense mechanisms
- •Suggested readings
- •23. Pneumonia
- •Etiology and pathogenesis
- •Pathology
- •Pathophysiology
- •Clinical features and initial diagnosis
- •Therapeutic approach: General principles and antibiotic susceptibility
- •Initial management strategies based on clinical setting of pneumonia
- •Suggested readings
- •24. Bacterial and viral organisms causing pneumonia
- •Bacteria
- •Viruses
- •Intrathoracic complications of pneumonia
- •Respiratory infections associated with bioterrorism
- •Suggested readings
- •25. Tuberculosis and nontuberculous mycobacteria
- •Etiology and pathogenesis
- •Definitions
- •Pathology
- •Pathophysiology
- •Clinical manifestations
- •Diagnostic approach
- •Principles of therapy
- •Nontuberculous mycobacteria
- •Suggested readings
- •26. Miscellaneous infections caused by fungi, including Pneumocystis
- •Fungal infections
- •Pneumocystis infection
- •Suggested readings
- •27. Pulmonary complications in the immunocompromised host
- •Acquired immunodeficiency syndrome
- •Pulmonary complications in non–HIV immunocompromised patients
- •Suggested readings
- •28. Classification and pathophysiologic aspects of respiratory failure
- •Definition of respiratory failure
- •Classification of acute respiratory failure
- •Presentation of gas exchange failure
- •Pathogenesis of gas exchange abnormalities
- •Clinical and therapeutic aspects of hypercapnic/hypoxemic respiratory failure
- •Suggested readings
- •29. Acute respiratory distress syndrome
- •Physiology of fluid movement in alveolar interstitium
- •Etiology
- •Pathogenesis
- •Pathology
- •Pathophysiology
- •Clinical features
- •Diagnostic approach
- •Treatment
- •Suggested readings
- •30. Management of respiratory failure
- •Goals and principles underlying supportive therapy
- •Mechanical ventilation
- •Selected aspects of therapy for chronic respiratory failure
- •Suggested readings
- •Index

17: Anatomic and physiologic aspects of neural, muscular, and chest wall interactions with the lungs
OUTLINE
Respiratory Control, 215
Organization of Respiratory Control, 215
Ventilatory Response to Hypercapnia and Hypoxia, 218
Ventilatory Response to Other Stimuli, 220
Respiratory Muscles, 220
Movement of gas into and out of the lungs requires the action of a pump capable of creating negative intrathoracic pressure, expanding the lungs, and initiating airflow with each inspiration, whereas expiration is largely passive under most circumstances. This cyclical pump-like action is provided by the respiratory muscles, including the diaphragm, working in conjunction with the chest wall. However, the muscles themselves have no intrinsic rhythmic activity in the way cardiac muscle does; they must be driven by rhythmic impulses provided by a “controller.”
This chapter focuses on the anatomic and physiologic features of the controlling system and the respiratory muscles to provide background for the discussion in Chapters 18 and 19. Those chapters discuss disorders affecting respiratory control, respiratory musculature, and the chest wall. Although much of the physiology and many of the clinical problems discussed here and in the next two chapters do not directly involve the lungs, they are so closely intertwined with respiratory function and dysfunction that they are appropriately considered in a textbook of pulmonary disease.
Respiratory control
Although the process of breathing is a normal rhythmic activity that occurs without conscious effort, it involves an intricate controlling mechanism at the level of the central nervous system (CNS). The CNS transmits signals to the respiratory muscles, initiating inspiration approximately 12 to 20 times per minute under normal circumstances. Remarkably, this controlling system is normally able to respond to varied needs of the individual, appropriately increasing ventilation during exercise and maintaining arterial blood gas parameters within a narrow range.

This section begins with a description of the structural organization of neural control of ventilation and proceeds to a consideration of how various stimuli may interact with and adjust the output of the respiratory controller. The ways the output of the controller can be quantified and how these techniques have proved useful in evaluating patients with a variety of clinical disorders are briefly discussed.
Organization of respiratory control
The basic organization of the respiratory control system is shown in Fig. 17.1. Crucial to this system is the CNS “generator.” Signals that originate from the generator travel down the spinal cord to the various respiratory muscles. The inspiratory muscles, the most important of which is the diaphragm, respond to the signals by contracting and initiating inspiration. This process is described later in more detail under the section “Respiratory Muscles.”
FIGURE 17.1 Schematic diagram showing organization of respiratory control
system. Dashed lines show feedback loops affecting respiratory generator. CNS,
central nervous system.
As a result of inspiratory muscular contraction, the diaphragm descends, the chest wall expands, and air flows down a pressure gradient from the mouth through the tracheobronchial tree to the alveolar spaces. Gas exchange in the distal parenchyma allows movement of O2 into the blood and release of CO2 from the blood into gas within the alveoli.
Although this sequence of events sounds relatively straightforward, it is regulated by an intricate and complicated feedback system that adjusts the output of the generator to achieve the desired effect. If the
Данная книга находится в списке для перевода на русский язык сайта https://meduniver.com/

response of the respiratory muscles to the generator’s signal is inadequate, as judged by a variety of respiratory “reflexes,” the generator increases its output to compensate for the lack of expected effect. If the arterial blood gas variables deviate from appropriate values, input from chemosensors for pH, O2, and CO2 to the respiratory generator is altered, ultimately affecting its output. In addition, input from other regions of the CNS, particularly the cerebral cortex and pons, can adjust the generator’s net output.
The respiratory generator
Considering the importance of the respiratory generator in this scheme of respiratory control, its anatomy and mode of action are described here. Much of the work clarifying the location of the respiratory generator involved animal experiments with transections at various levels of the CNS and assessment of the effects on ventilation. Because transection between the brain and brainstem does not significantly alter ventilation, the generator apparently resides somewhere at the level of the brainstem or lower and does not require interaction with higher cortical centers. When transections are made at various points within the brainstem, the breathing pattern is substantially altered, but ventilation is not eliminated. Only when a transection is made between the medulla and the spinal cord does ventilation cease, indicating that the respiratory generator resides within the medulla. In humans and other mammals, the collection of neurons in the ventrolateral medulla that appears to be essential for respiratory pattern generation is termed the pre-Bötzinger complex.
A central respiratory generator within the medulla controls activity of the respiratory muscles.
Although the respiratory center (or generator) has been referred to as a single region, more than one network of neurons within the medulla are involved in initiating and coordinating respiratory activity. According to a popular model, one group of neurons is responsible for initiating inspiration and regulating its speed as a result of the intensity of neuronal activity; another group of neurons controls “switching off” inspiration and hence determines the onset of expiration.
Therefore, there are two aspects of ventilatory control: (1) the degree of inspiratory drive or central inspiratory activity (which regulates the inspiratory flow rate), and (2) the timing mechanism (which controls the termination of inspiration). These two determining factors act in concert to set the respiratory rate and tidal volume and thus the minute ventilation and specific pattern of breathing.
Input from other regions of the central nervous system
Even though the medullary respiratory center does not require additional input to drive ventilation, it does receive other information that contributes to a regular pattern of breathing and more precise ventilatory control. For example, input from the pons appears to be necessary for a normal, coordinated breathing pattern. When the influence of the pons is lost, irregularities in the breathing pattern ensue.
In addition to pathways involved in the “automatic” or involuntary control of ventilation, the cerebral cortex exerts a conscious or voluntary control over ventilation. Cortical overriding of automatic control can be seen with either voluntary breath-holding or voluntary hyperventilation. Its usefulness is readily apparent in a person’s need for voluntary control of breathing during such activities as speaking, eating, and swimming. Interestingly, the automatic control of ventilation may be disturbed while conscious control remains intact. In these cases, during wakefulness the cerebral cortex exerts sufficient voluntary control over ventilation to maintain normal arterial blood gas values. During periods when the patient is dependent on automatic ventilatory control (e.g., during sleep), marked hypoventilation or apnea may occur. This rare condition, called congenital central hypoventilation syndrome, has also been known as Ondine’s curse, after a mythologic tale in which the suitor of Neptune’s daughter was cursed to lose
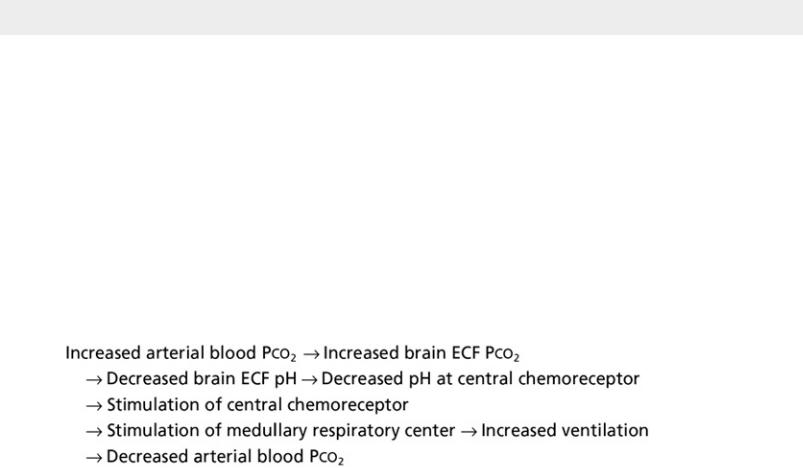
automatic control over all bodily functions when he fell asleep. Defects in the PHOX2b gene mapped to chromosome 4p12 have been identified in most cases. PHOX2b encodes a highly conserved domain for transcription factors important in neural development. Further research into the pathogenesis of this condition undoubtedly will lead to better understanding of the mechanisms of normal ventilatory control.
Chemoreceptors
Maintenance of normal arterial blood gas parameters is the ultimate goal of ventilatory control, and an important feedback loop adjusts respiratory center output if these parameters are not maintained (see Fig. 17.1). Elevation of PCO2 (hypercapnia) and depression of PO2 (hypoxemia) will both stimulate ventilation. In each case, one or more chemoreceptors detect alterations in PCO2 or PO2 and accordingly vary their input to the medullary respiratory center.
Changes in PCO2 are sensed primarily at a central chemoreceptor in the medulla.
The primary sensor regulating CO2 is located near but separate from the medullary respiratory center on the ventrolateral surface of the medulla and is called the central chemoreceptor. The central chemoreceptor does not respond directly to blood PCO2 but rather to the pH of the extracellular fluid (ECF) surrounding the chemoreceptor. The pH, in turn, is determined by the concentration of hydrogen (H+) and bicarbonate (HCO3−) ions, as well as PCO2 in the ECF (see Appendix C for further discussion). The composition of cerebrospinal fluid (CSF) and brain ECF is influenced by the permeability properties of the blood-brain barrier: CO2 diffuses freely, whereas the movement of either H+ or HCO3− from blood to brain ECF is constrained. Thus, blood CO2 level indirectly affects the central chemoreceptor by affecting the composition of the ECF. The feedback loop for changes in PCO2 can be summarized as follows:
There are two primary sensors for O2, the carotid body and aortic body chemoreceptors, which are both located in peripheral blood vessels, not the CNS. The carotid chemoreceptors, which are quantitatively much more important than the aortic chemoreceptors, are located just beyond the bifurcation of each common carotid artery into the internal and external carotid branches. The aortic chemoreceptor is found between the pulmonary artery and the aortic arch. These chemoreceptors are sensitive to changes in PO2, with hypoxia stimulating chemoreceptor discharge. In adults, the peripheral carotid body chemoreceptors also have a role in sensing PCO2. Under normoxic conditions, the peripheral chemoreceptors play a much less important regulatory function than the central chemoreceptors for maintaining PCO2. However, arterial hypoxemia increases the sensitivity of the peripheral PCO2 receptors, so if hypoxemia and hypercarbia are both present, the carotid body chemoreceptor will be maximally stimulated to increase ventilation. Peripheral chemoreceptor discharge is transmitted back to the CNS by
cranial nerves: the glossopharyngeal nerve (cranial nerve IX) in the case of the carotid bodies and the
Данная книга находится в списке для перевода на русский язык сайта https://meduniver.com/

vagus nerve (cranial nerve X) for the aortic body chemoreceptor. The information ultimately is transmitted to the medullary respiratory center so that its output is augmented.
The major sensors for PO2 are the peripheral (carotid and aortic body) chemoreceptors.
Input from other receptors
In addition to chemoreceptor effects, input from receptors in the lung (including the airways), which are carried via the vagus nerve to the CNS, can influence ventilation. Stretch receptors located within the smooth muscle of airway walls respond to changes in lung inflation. As the lung is inflated, receptor discharge increases. In diving animals, this stretch receptor reflex (the Hering-Breuer reflex) is responsible for apnea that occurs as a result of lung inflation. In contrast, conscious human adults do not readily demonstrate the Hering-Breuer reflex, but in human infants the reflex is strong enough to limit tidal volumes and control the respiratory rate, preventing overinflation of the lungs in early infancy. Presumably, stretch receptors contribute to switching off inspiration and initiating expiration after a critical level of inspiratory inflation has been reached.
Irritant receptors located superficially along the lining of airways may initiate tachypnea, usually in response to some noxious inhaled stimulus such as a chemical or irritating dust. Juxtacapillary (or J) receptors are found within the pulmonary interstitium, adjacent to capillaries. One of their effects is to cause tachypnea, and they may be responsible for the respiratory stimulation caused by inflammatory processes or accumulation of fluid within the pulmonary interstitium.
Receptors in the chest wall, particularly in the intercostal muscles, appear to play a role in fine-tuning ventilation. The muscle spindles are part of a reflex arc that adjusts the output of respiratory muscles if the desired degree of muscular work has not been achieved. When a mismatch occurs between the output from the CNS controller and the amount of “stretch” sensed by these receptors, feedback from the receptors is involved in causing dyspnea. For example, in the patient with severe emphysema and lung hyperinflation, the increased output from the brain does not produce an “appropriate” change in lung inflation. Because the neural output is not matched by the resultant lung expansion, feedback is transmitted through the stretch receptors in the chest wall to the brain, and the patient experiences dyspnea. The precise mechanisms of these pathways are incompletely understood.
Ventilatory response to hypercapnia and hypoxia
Two of the stimuli for ventilation that have been best studied are well-defined chemical stimuli: hypercapnia and hypoxia. As discussed above, hypercapnia is sensed primarily but not exclusively by the central chemoreceptor, and the stimulus appears to be the pH level of brain ECF. In contrast, hypoxia stimulates ventilation by acting on peripheral chemoreceptors, carotid much more than aortic.
When arterial PO2 is held constant, ventilation increases by approximately 3 L/min for each millimeter of mercury rise in arterial PCO2 in adults. This relatively linear response, the magnitude of which varies considerably among individuals, is shown in Fig. 17.2. Also shown is the effect of PO2 on the response to increments in PCO2. At a lower PO2, the response to hypercapnia is heightened.

FIGURE 17.2 Ventilatory response to progressive elevation of PCO2 in a normal individual. Solid line (A) shows response when simultaneous PO2 is high (hyperoxic conditions). Dashed line (B) shows heightened response when
simultaneous PO2 is low (hypoxic conditions).
With chronic hypercapnia, the ventilatory response to further increases in PCO2 is diminished. The reason for the blunted CO2 responsiveness is relatively straightforward. When CO2 retention persists for days, the kidneys compensate for the more acidic pH by excreting more hydrogen ions and less bicarbonate, and the levels of bicarbonate rise in both plasma and brain ECF. The elevated bicarbonate level can buffer any acute changes in PCO2 more successfully, so that the brain ECF pH value changes less for any given increment in PCO2.
Ventilatory responsiveness to CO2 is blunted in patients with chronic hypercapnia.
With hypoxemia, the same linear relationship does not exist between alterations in partial pressure and ventilation. Rather, the ventilatory response is relatively small until PO2 falls to approximately 60 mm Hg, below which the rise in ventilation is much more dramatic (Fig. 17.3). The curvilinear relationship between PO2 and ventilation can be made linear if ventilation is plotted against O2 saturation instead of partial pressure (Fig. 17.4). However, despite the linear relationship between ventilation and O2 saturation, it is the partial pressure of O2, not the oxygen content or saturation, that is sensed by the chemoreceptor.
Данная книга находится в списке для перевода на русский язык сайта https://meduniver.com/

FIGURE 17.3 Ventilatory response to progressively decreasing PO2 with PCO2 kept constant in a normal individual. Ventilation does not rise significantly until PO2 falls to approximately 60 mm Hg.

FIGURE 17.4 Ventilatory response to hypoxia, plotted using O2 saturation rather than PO2. Relationship between ventilation and O2 saturation during progressive hypoxia is linear. Solid line (A) shows response when measured at normal PCO2. Dashed line (B) shows augmented response at elevated PCO2.
PCO2 also has an effect on a patient’s response to hypoxia. The sensitivity to hypoxia is increased as PCO2 is raised and is decreased as PCO2 is lowered (see Fig. 17.4). This feature is important to consider when testing for responsiveness to hypoxia. As the patient hyperventilates in response to a low PO2, PCO2 drops, and ventilation is stimulated less than it would be if PCO2 were unchanged. Therefore, PCO2 should be kept constant so that the condition for testing actually is “isocapnic” hypoxia.
When the clinician suspects a disorder of ventilatory control, the ventilatory response to hypercapnia or hypoxia can be quantified. However, the responses to these stimuli vary widely even in seemingly normal individuals, presumably due to genetic variation. This fact must be taken into account in the interpretation of ventilatory response data.
Ventilatory response to other stimuli
One of the most important times for a rapid and appropriate increase in ventilation is in response to a change in metabolic requirements. For example, with the metabolic needs of exercise, a normal individual can increase ventilation from a resting value of 5 L/min to 60 L/min or more, without any demonstrable change in arterial blood gas values. According to one popular theory, the initial rapid increase in ventilation at the onset of exercise (i.e., before PaO2 or PaCO2 begin to change) is due to a neural stimulus, although the origin is not clear. After the initial rapid augmentation in ventilation, there occurs a later and slower rise that probably is due to a bloodborne chemical stimulus. However, many questions about the remarkably precise way ventilation responds to the demands of exercise remain unanswered.
Данная книга находится в списке для перевода на русский язык сайта https://meduniver.com/