
Книги по МРТ КТ на английском языке / Functional Neuroimaging in Child Psychiatry Ernst 1 ed 2000
.pdf
Table 10.1 (cont.)
Study |
Subjects |
|
Controls |
|
|
|
|
|
|
|
|
|
|
|
|
|
|
|
|
|
Number |
Age |
Number |
Age |
Diagnostic |
Testing |
|
||
|
(sex) |
(years) |
(sex) |
(years) |
criteria |
condition |
Results |
||
|
|
|
|
|
|
|
|
|
|
Chugani et al. |
Healthy |
2±15 |
Healthy siblings: |
2±14 |
|
ADI-R, DSM IV, |
Sedated; controls |
Whole-brain serotonin synthesis capacity showed |
|
(1999a) |
autistic: |
|
6M, 2F |
|
|
CARS, GARS |
sedated |
diVerent changes with age in autistic compared with |
|
|
24M, 6F |
|
Epilepsy: 9M, 7F |
3 months to |
|
Sedated (4/8) |
nonautistic group |
||
|
|
|
|
|
14 years |
|
|
|
|
Metabolites measured with MRS |
|
|
|
|
|
|
|
||
Minshew et al. |
11M |
12±36 |
11M |
12±36 |
|
DSM-III-R, |
Not sedated |
Decreased levels of phosphocreatine and esteriWed |
|
(1993) |
|
|
|
|
|
|
ADI, ADOS |
|
ends in dorsal prefrontal cortex |
Hashimoto et al. |
20M, 8F |
2±12 |
16M, 9F |
2±13 |
|
DSM-III-R |
Sedated |
No diVerences in N-acetylaspartate/choline ratio in |
|
(1997) |
|
|
|
|
|
|
|
|
voxel located in right parietal region; no lactate |
|
|
|
|
|
|
|
|
|
detected |
Chugani et al. |
8M, 1F |
3±12 |
Healthy siblings: |
8±14 |
|
DSM-IV, CARS, |
Sedated; controls |
Lower N-acetylaspartate in cerebellum; lactate |
|
(1999b) |
|
|
4M, 1F |
|
|
GARS |
not sedated |
detected in frontal lobe in 1 autistic boy |
|
|
|
|
|
|
|
|
|
|
|
|
|
|
|
|
|
|
|
|
|
Notes:
ABC, autistic behavior checklist; ASIEP, autistic symptom inventory educational pro®le; ADI, Autism Diagnostic Index; ADOS, Autism Diagnostic Observation Scale; CARS, Childhood Autism Rating Scale; DSM-III-R, Diagnostic and Statistical Manual, 3rd edn revised (American Psychiatric Association, 1987); GARS, Gilliam Autism Rating Scale; ICD10, 10th draft of the International Classi®cation of Diseases (World Health Organization, 1987); ICDS, interview for childhood disorders and schizophrenia.
a Indicates overlap in subject samples (diVerent analyses of the same or overlapping sample).
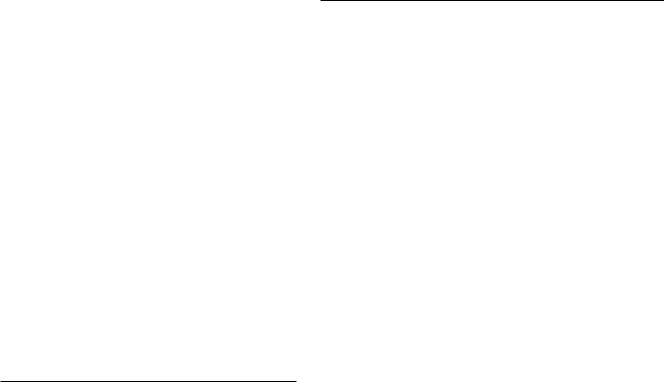
Heterogeneity in etiology of autism
Autism may be a component feature of a number of disorders, such as fragile X syndrome (Payton et al., 1989; Piven et al., 1991), phenylketonuria (Friedman, 1969), tuberous sclerosis complex (Smalley et al., 1992), Rett syndrome (Hagberg, 1985), prenatal viral infection (reviewed in Lotspeich and Ciaranello, 1993), and several other conditions (reviewed in Ciaranello and Ciaranello, 1995). Manifestations vary according to developmental level and chronologic age, and there is marked heterogeneity of the behavioral characteristics among autistic individuals. Several studies have attempted to identify subtypes of symptom clusters. ClassiWcation systems of autistic subtypes generally are related to degree of severity and include intellectual functioning (Volkmar et al., 1987), social interactions (Wing and Gould, 1979), and behaviorally homogeneous subtypes (Sevin et al., 1995).
Functional imaging studies in autistic subjects
It is diYcult to scan young children and to obtain appropriate age-matched controls; consequently, the majority of functional imaging studies of autistic subjects have employed adolescents and adults. In addition, the studies have overwhelmingly concentrated upon high-function- ing autistic subjects since these individuals are able to cooperate with imaging procedures. This approach seriously limits our ability to probe the developmental changes in autistic brain. Moreover, adults with autism have an extended history of having undergone a variety of behavioral, sensory, dietary, herbal, and pharmacologic interventions. The eVects of these treatments upon brain function are unknown and diYcult to control. In addition, there may be chronic changes that occur with age in this disorder. Evidence for degenerative changes in cerebellar nuclei were suggested in older subjects in the neuropathology studies of Bauman and Kemper (1994).
Focal and global brain alterations in glucose metabolism
Increased global brain glucose metabolism in autism
The measurement of 2-deoxy-2-[18F]-Xuoro-d-glucose (FDG) with positron emission tomography (PET) has proved to be a valuable tool in identifying regional and global abnormalities in many neurologic conditions. The impact of this method toward the understanding of autism has been less clear. In the Wrst study of glucose metabolism in autism, Rumsey et al. (1985) reported diVusely increased
Autism 175
glucose metabolism by approximately 20% in a group of 10 autistic men compared with 15 healthy genderand agematched control subjects. The Wnding of globally increased glucose metabolism has not been replicated in subsequent FDG PET studies reported (de Volder et al., 1987; Herold et al., 1988; Siegel et al., 1992). However, there were methodologic diVerences in the subsequent studies; therefore, diVerences in global glucose metabolism in autistic adults cannot be discounted. For example, Herold et al. (1988) compared six male autistic subjects with six healthy males and two females. Similarly, Siegel et al. (1992) compared autistic adults (12 males, four females; 17±38 years) and normal controls (19 males, seven females; mean age 27 years) mixed for gender and found no diVerence in global glucose metabolism. Since there are gender diVerences in glucose metabolism on the same order of magnitude of those Rumsey et al. reported between autistic and normal men (Baxter et al., 1987), the inclusion of females in control groups could mask a true global increase in glucose metabolism. De Volder et al. (1987) reported no diVerences in global glucose metabolism in 18 autistic children (11 male, seven female aged 2±18 years) compared with a control group that comprised children (three normal children aged 7, 14, and 15 years; three children with unilateral pathology aged 9, 12, and 12.5 years) with various brain pathologies, as well as 15 adults (mean age 22 years). Few conclusions can be drawn from the de Volder study since glucose metabolism shows marked changes with age (Chugani et al., 1987; see below).
Regional brain glucose metabolism alterations in autism and related disorders
Horwitz et al. (1988) added four male autistic subjects to the series reported by Rumsey et al. (1985) and showed that the global brain glucose metabolic rate (CMRGlu) was 12% higher in the autistic group, a diVerence that was statistically signiWcant. In addition, Horwitz et al. (1988) performed a correlation analysis that showed signiWcantly fewer positive correlations between frontal and parietal cortices, with the most notable discrepancy found between the left and right inferior frontal regions. Furthermore, the thalamus and basal ganglia also showed less correlation with frontal and parietal cortices in the autistic group compared with the controls.
Focal abnormalities of glucose metabolism have been reported in a number of other studies in which global brain glucose metabolism was not addressed. Heh et al. (1989) studied glucose metabolism in the cerebellum based upon neuropathologic data showing fewer Purkinje and granule cells in the cerebellum (Bauman and Kemper, 1985; Ritvo et al., 1986) and vermal cerebellar hypoplasia measured by

176D. C. Chugani
magnetic resonance imaging (MRI) (Courchesne et al., 1988). However, Heh et al. (1989), showed no signiWcant diVerence in mean CMRGlu for cerebellar hemispheres or vermal lobes VI and VII in autistic subjects (Wve males and two females; 19±36 years ) compared with control subjects (seven males, one female; 20±35 years). Schifter et al. (1994) studied a heterogeneous group of children (nine males, four females; 4±11 years) with autistic behavior coexisting with seizures, mental retardation, and neurologic abnormalities. Visual analysis of the FDG PET scans revealed that 5 of the 13 subjects had focal abnormalities located in diVerent brain regions for each patient. Regions showing hypometabolism included right cerebellum and left temporal/parietal/occipital cortices; right parietal cortex, bilateral thalamus and left occipital cortex; right parietal and left temporal/parietal cortices; right parietal/occipital and left occipital cortices; and bilateral temporal lobes.
Buchsbaum et al. (1992) applied a visual continuous performance task, which was associated with greater right than left hemisphere metabolism in autistic subjects (Wve males, two females; 19±36 years) than in their normal control subjects (13 males; mean age 24 years). Siegel et al. (1992) studied 16 high-functioning autistic adults (12 males, four females; 17±38 years) and 26 normal controls (19 males, seven females; mean age 27 years) and reported that autistic subjects had a left greater than right anterior rectal gyrus asymmetry, as opposed to the normal right greater than left asymmetry in that region. The autistic group also showed low glucose metabolism in the left posterior putamen and high glucose metabolism in the right posterior calcarine cortex. The same group (Siegel et al., 1995) studied glucose metabolism in 15 adults with a history of infantile autism (12 males, three females; aged 17±38 years, mean 24 years; 15 of 16 subjects previously reported by Siegel et al. (1992)) and reported that autistic subjects showed abnormal thalamic glucose metabolism; correlations of task performance with pallidal metabolism suggested subcortical dysfunction during the attentional task in autism. Recently, Haznedar et al. (1997) performed MRI and glucose PET scans on seven high-functioning autistic patients (Wve males, two females; mean age 24.3 years) and seven sexand age-matched normal adults. Right anterior cingulate was signiWcantly smaller in relative volume and was metabolically less active in the autistic patients than in the normal subjects. However, these data were not corrected for partial volume eVects (HoVman et al., 1979), and the apparent decrease in glucose metabolism may be secondary to the reported volume decrease.
An association of autism in children with a history of infantile spasms has been long recognized (Riikonen and
Amnell, 1981). Chugani et al. (1996b) reported that 18 children (seven males, 11 females; 10 months to 5 years of age) from a total of 110 children with a history of infantile spasms showed bilateral temporal lobe glucose hypometabolism on PET, with normal MRI scans. Long-term outcome data was obtained for 14 of the 18 children; 10 of the 14 children met DSM-IV criteria for autism. All 14 children had continued seizures and mental retardation. Two temporal lobe regions, superior temporal gyrus and hippocampus, showed signiWcant hypometabolism compared with that in age-matched controls. These observations are relevant not only because histologic studies of brain tissue at autopsy from autistic subjects show abnormalities in hippocampus (Bauman and Kemper, 1994) but also because recent studies using volumetric MRI in patients with fragile X syndrome have found abnormalities in hippocampus (increased volume) and superior temporal gyrus (decreased volume) (Reiss and Freund, 1994).
Focal and global brain alterations in resting blood Xow
A number of studies of autistic subjects measuring cerebral blood Xow with single photon emission computed tomography (SPECT) can be found in the literature and report a variety of global and focal abnormalities. George et al. (1992) reported global hypoperfusion in the resting state in adult autistic men with seizures (four males; 22±34 years) compared with control subjects (two males, two females; 25±32 years). George et al. (1992) further observed pronounced hypoperfusion in the frontotemporal cortices, whereas McKelvey et al. (1995) localized most consistent hypoperfusion to the vermis and the right cerebellar hemisphere in three adolescent autistic subjects (two males, one female; 14±17 years). Mountz et al. (1995) also reported hypoperfusion in autistic subjects (Wve males, one female; 9±21 years) compared with the control group (Wve males, two females; 6±20 years) but localized it primarily to the left temporoparietal and the right anterior temporal region.
Zilbovicius et al. (1992) measured regional cerebral blood Xow with SPECT and xenon-133 in 21 children (12 boys, nine girls; 5±11 years, mean 7.4) with autism according to DSM-III-R criteria. Five cortical brain areas including frontal, temporal, and sensory association cortices were examined. The group with autism showed no cortical regional abnormalities compared with an age-matched group of 14 nonautistic children with slight-to-moderate language disorders.While the autistic subjects in this study were sedated, the control group (those with language disorders) were not. Zilbovicius et al. (1995) also studied cerebral blood Xow in preschool autistic children in a

Autism 177
longitudinal study. Five autistic children (three males, two females) were studied at the age of 3±4 years and 3 years later and were compared with two age-matched comparison groups of nonautistic children (Wve children ages 3±4 years, and seven aged 6±12 years) with normal development. These investigators reported frontal hypoperfusion in the autistic children at ages 3±4 years, but not at the ages of 6±7; they concluded that these results indicated a delayed frontal maturation in childhood autism. Chiron et al. (1995) compared blood Xow in 18 autistic children (14 males, four females; 4±14 years) with 10 control subjects (Wve males, Wve females; 4±16 years) and found blood Xow was greater in the left hemisphere in control subjects but greater in the right in autistic patients. All but one of the autistic subjects was sedated with intrarectal pentobarbital (pentobarbitone) and, in some cases, intramuscular droperidol, while only two of the ten control subjects were sedated. While barbiturates have been reported to decrease cerebral metabolism in adults (Theodore et al., 1986), Chiron et al. (1992) used 133Xe SPECT to show that cerebral blood Xow changes induced by pentobarbital were not statistically signiWcant in children.
Blood Xow changes during the performance of tasks
In a functional mapping study using H215O PET, Happé et al. (1996) applied a ªtheory of mindº task that required attributing mental states to the characters of a narrative. The statistical parametric mapping analysis showed that the Asperger's group (Wve males, 20±27 years) showed a slightly diVerent location of activation in inferior prefrontal cortex (Brodmann area 9 instead of 8) compared with the normal control group (six males; 24±65 years).
Müller et al. (1999) studied auditory perception and receptive and expressive language in Wve high-functioning autistic adults (four males, one female; 18±31 years) compared with Wve normal men (23±30 years) using an H215O activation paradigm. Scans were performed at rest, and while subjects listened to tones, listened to short sentences, repeated short sentences, and generated sentences. Analyses of peak activations revealed reduced or reversed dominance for language perception in temporal cortex, and reduced activation of auditory cortex and the cerebellum during acoustic stimulation in the autistic group. Data from the four autistic men and Wve normal men were reanalysed (Müller et al., 1998) to examine three predetermined regions of interest ± dentate nucleus of the cerebellum, thalamus, and Brodmann area 46 ± based upon serotonin (5-HT) synthesis studies showing abnormalities in these three regions in autistic boys (Chugani et al., 1997). The results of this study showed that the dorso-
lateral prefrontal cortex (area 46) and thalamus in the left hemisphere and the right dentate nucleus showed less activation in the autistic men than in the control group for sentence generation. In contrast, with sentence repetition, increases in blood Xow were signiWcantly larger in left frontal cortex and right dentate nucleus in the autistic subjects than the control group. These data suggest that left frontal cortex, left thalamus, and right dentate nucleus showed atypical functional changes with language tasks in high-functioning autistic men.
Because of the small numbers of subjects, all of the functional mapping studies so far performed should be considered pilot studies. However, this is a promising approach for high-functioning subjects who are able to cooperate with the performance demands of this type of study.
DiVerences in brain metabolites
Magnetic resonance spectroscopy (MRS) is a technique that can be used to detect and quantify biochemicals in living brain using MRI technology. Phosphorus-31-MRS can be used to assess energy metabolism by quantifying steady-state levels of phosphocreatine, adenosine triphosphate (ATP), adenosine diphosphate (ADP), and inorganic orthophosphate (for reviews see Chapter 1 and Bottomley and Hardy, 1989). In addition, phospholipid metabolism can be assessed by the measurement of phosphomonoesters and phosphodiesters. Proton MRS (1H MRS; for review see Moore, 1998) can be used to measure the levels of a number of brain metabolites, including N-acetylaspartate (NAA), which is a putative neuronal marker that is an indicator of neuronal function/viability, lactate, which is a metabolite of energy metabolism not normally detectable in brain but detected in some pathologic states (Jenkins et al., 1993), choline, creatine, and several neurotransmitters such as gamma-aminobutyric acid (GABA) and glutamate.
Using 31P MRS to measure phosphorus metabolites in dorsal prefrontal cortex in 11 male autistic adolescents and adults (aged 12±36 years) and 11 age-, gender-, and socioe- conomic-matched normal controls, Minshew et al. (1993) found decreased levels of phosphomonoesters, increased levels of phosphodiesters, and decreased levels of ATP. This group suggested that their results indicated decreased synthesis and/or increased breakdown of membrane phospholipids, with increased ATP consumption, perhaps related to abnormal dendritic integrity.
Hashimoto et al. (1997) performed 1H MRS in occipital cortex in 28 patients with autism (20 males, eight females; 2±12 years), 28 age-matched patients with mental retardation (22 males, six females; 2±13 years) and 25 agematched healthy children (16 males, nine females; 2±13

178D. C. Chugani
years). Peaks for NAA, choline, and creatine, but not lactate, were observed in each group. The NAA/choline ratio was lower in patients with mental retardation than in patients with autism and in controls, but there were no diVerences in the NAA/choline ratios between patients with autism and controls. Chugani et al. (1999b) performed 1H MRS in nine autistic children (eight males, one female; 3±12 years, mean age 5.7 years) and Wve of their healthy nonautistic siblings (four males, one female; 6±14 years, mean age 9 years). Voxels of 8ml were sampled in frontal cortex, temporal cortex, and cerebellum, and concentrations of NAA and lactate were measured using the cerebral water signal as an internal standard. Lactate, which is not normally detected in brain, was detected in one of the nine autistic children studied, but in none of the controls. These data are consistent with altered energy metabolism in some autistic children. NAA was signiWcantly lower in cerebellum in the autistic group than in the sibling group, while mean NAA values for frontal lobe and temporal lobe did not signiWcantly diVer between the groups. The Wnding of lower NAA in cerebellum in the autistic group is consistent with neuropathology reports of decreased numbers of Purkinje cells and granule cells in cerebellar cortex from autistic individuals (Bauman and Kemper, 1994).
These promising preliminary MRS Wndings in autistic subjects suggest that further studies of autism with this technique are warranted. Studies reported so far used the single-voxel method and were limited in the regions of the brain that could be sampled in a scanning session. Newer spectroscopic imaging techniques now allow sampling of the entire brain within an acceptable scan acquisition time.
Focal and global brain alterations in neurotransmission
Studies investigating alterations in neurotransmitters, hormones, and their metabolites in the blood, cerebrospinal Xuid (CSF), and urine of autistic patients have been numerous and have provided some evidence for the potential involvement of several neurotransmitters in autism (for review see Anderson, 1994). Furthermore, given there is evidence for dysfunction in widely distributed brain regions in autism (Minshew et al., 1997), the monoamine neurotransmitters are interesting candidates because of their widespread modulatory role in the brain. To this purpose, functional imaging has been used to examine the role for two monoamine transmitters, dopamine (Ernst et al., 1997) and serotonin (Chugani et al., 1997, 1999a), in autism.
Functional imaging evidence for dopaminergic dysfunction in autism
Ernst et al. (1997) studied 14 medication-free autistic children (eight males, six females; mean age 13 years) and 10 healthy children (seven males, three females; mean age 14 years) with 18F Xuorodopa (F-DOPA) using PET. F-DOPA is a precursor of dopamine, which is taken up, metabolized, and stored by dopaminergic terminals. Ernst and colleagues calculated the ratios of F-DOPA activity (as the region of interest/occipital cortex) measured between 90 and 120min following tracer administration. Regions sampled were the caudate, putamen, midbrain, and lateral and medial anterior prefrontal regions (all regions rich in dopaminergic terminals), and occipital cortex (a region poor in dopaminergic terminals). They reported a 39% reduction of the F-DOPA ratio in the anterior medial prefrontal cortex/occipital cortex in the autistic group. There were no signiWcant diVerences in any of the other regions measured. These authors suggested that decreased dopaminergic function in prefrontal cortex may contribute to the cognitive impairment seen in autism.
Functional imaging evidence for serotonergic dysfunction in autism
Although there is evidence for the potential involvement of several neurotransmitters in autism, the most consistent abnormal neurotransmitter Wndings involve serotonin. Schain and Freedman (1961) Wrst reported increased blood serotonin in approximately one third of autistic patients. Studies of the serotonin metabolite 5-hydroxyindoleacetic acid (5-HIAA) in CSF have failed to demonstrate consistent abnormalities of central serotonergic tone (for review see Anderson et al., 1994). Pharmacologic treatments that decrease serotonergic neurotransmission, such as tryptophan depletion, have been reported to result in an exacerbation of symptoms in autistic subjects (McDougle et al., 1996a). Conversely, administration of serotonin-reuptake inhibitors appear to result in improvement of compulsive symptoms, repetitive movements, and social diYculties in autistic adults (Cook et al., 1992; Gordon et al., 1993; McDougle et al., 1996b). Finally, a role for serotonin in autism is also suggested by the recent Wnding that an ineVeciently transcribed serotonin transporter polymorphism showed signiWcantly higher incidence in autistic individuals and their families (Cook et al., 1997).
Chugani et al. (1997, 1999b) have applied '-[11C]- methyl-l-tryptophan ([11C]-AMT) as a PET tracer in autistic subjects. It is used to follow serotonin synthesis with PET (Diksic et al., 1990) because it is an analog of tryptophan, the precursor for serotonin synthesis. Following the administration of labeled or unlabeled AMT in rats, the
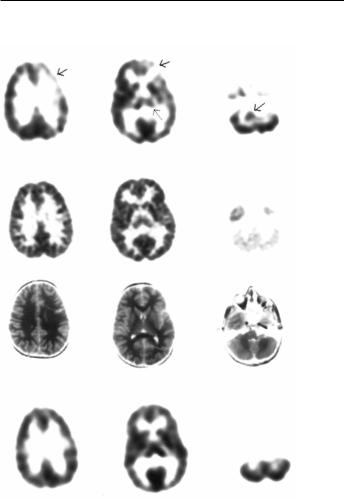
Autism 179
synthesis of '-methylserotonin (AM-5HT) in brain has been demonstrated by high-pressure liquid chromatography (Missala and Sourkes, 1988; Diksic et al., 1990).
Synthesis of AM-5HT in brain has been localized to serotonergic neurons and nerve terminals by combined autoradiography detecting [3H]-AM-5HT and tryptophan hydroxylase immunocytochemistry at the electron microscopic level (Cohen et al., 1995). Furthermore, [3H]-AM- 5HT present in nerve terminals could be released by K1-induced depolarization, suggesting that this tracer is stored within the releasable pool of serotonin (Cohen et al., 1995). Since AM-5HT, unlike serotonin, is not a substrate for the degradative enzyme monoamine oxidase (Missala and Sourkes, 1988), accumulation of AM-5HT occurs in serotonergic terminals. In addition, AMT, unlike tryptophan, is not incorporated into protein in signiWcant amounts (Madras and Sourkes, 1965; Diksic et al., 1990). These properties of AMT make it a suitable tracer substance for the measurement with PET of serotonin synthesis in vivo in humans (Muzik et al., 1997; Nishizawa et al., 1997; Chugani et al., 1998).
Chugani et al. (1997) used [11C]-AMT and PET to study healthy, seizure-free children with autism (seven males, one female; 4±11 years) and their healthy nonautistic siblings (four males, one female; 8±14 years). All of the autistic subjects and three of the Wve siblings were sedated with pentobarbital or midazolam. Gross asymmetries of [11C]- AMT standard uptake value (SUV) in frontal cortex, thalamus, and cerebellum were visualized in seven autistic boys, but not in the one autistic girl studied nor in four of Wve siblings (Fig. 10.1a). Decreased [11C]-AMT accumulation was seen in the left frontal cortex and thalamus in Wve of seven autistic boys. This was accompanied by an elevated [11C]-AMT accumulation on the right in the cerebellum. The region of increased tracer accumulation in the cerebellum appeared to be in the dentate nucleus, based upon coregistration with the MRI. In the remaining two autistic boys, [11C]-AMT accumulation was decreased in the right frontal cortex and thalamus and elevated in the left dentate nucleus. No asymmetries were seen in the frontal cortex or thalamus of the sibling group (Fig. 10.1d); however, one sibling showed an increased [11C]-AMT accumulation in the right dentate nucleus. Interestingly, this boy had a history of calendar calculation and he ritualistically lined up his toys, behaviors commonly seen in autistic children. The overall diVerence in asymmetry scores between the autistic boys and their siblings was found to be statistically signiWcant, and regional asymmetry scores in the frontal cortex and thalamus were also found to diVer signiWcantly. The speciWcity of these abnormalities in serotonin synthesis was apparent when comparing the
(a) Autistic boy (6.8 years of age)
(b)
(c )
(d ) Male sibling (9.4 years of age)
Fig. 10.1. Scans for an autistic boy aged 6.8 years (a±c) and his brother aged 9.4 years (d). (a), (d) PET images using '-[11C]- methyltryptophan ([11C]-AMT); (b) PET image using [18F]-
Xuorodeoxyglucose scan; (c) MRI scan. Arrows denote decreased [11C]-AMT accumulation in left frontal cortex and left thalamus and increased [11C]-AMT accumulation in right dentate nucleus in the autistic child (left side of image is the right side of the brain).
[11C]-AMT scans with the FDG PET and MRI scans, both of which were normal by visual examination in the children studied (Fig. 10.1b,c).
Chugani et al. (1999a) also measured whole-brain serotonin synthesis capacity in autistic and nonautistic children at diVerent ages using [11C]-AMT and PET. Global brain values for serotonin synthesis capacity were obtained for 30 healthy, seizure-free autistic children (24 males, six females; 2±15 years), eight of their healthy nonautistic siblings (six males, two females; 2±14 years), and 16 epileptic children without autism (nine males, seven females; 3 months to 13 years). Children in the epilepsy group had medically intractable seizures, but patients with multiple

180 D. C. Chugani
anatomic brain lesions (e.g., tuberous sclerosis) were not included. Epileptic patients were taking at least one anticonvulsant medication. All of the autistic and epileptic subjects and four of the eight siblings were sedated with pentobarbital or midazolam. For nonautistic children, serotonin synthesis capacity was $200% of adult values until the age of 5 years and then declined toward adult values. Serotonin synthesis capacity declined at an earlier age in girls than in boys. In autistic children, serotonin synthesis capacity increased gradually between the ages of 2 years and 15 years to values 1±1.5 times adult normal values and showed no gender diVerence. These data suggest that humans undergo a period of high brain serotonin synthesis capacity during childhood, and that this developmental process is disrupted in autistic children. Serotonin synthesis capacity was estimated in four highfunctioning autistic adults (three males, one female; mean age 26.5 years; mean full scale IQ 70) (Chugani et al., 1996a). Values of serotonin synthesis capacity for diVerent brain regions in one autistic woman studied fell within the range of values measured in Wve normal adult females. Comparisons made between autistic men and control men (n5 5) showed that mean serotonin synthesis capacity values were signiWcantly higher for all brain regions in the autistic group. Interestingly, clomipramine, a tricyclic nonselective serotonin-uptake inhibitor, has been shown to beneWt autistic adults (Gordon et al., 1992, 1993; McDougle et al., 1992), whereas treatment of eight autistic children with clomipramine resulted in a worsening of behavior in six of the children, including increased tantrums, irritability, aggression, self-injury, and crying spells (Sanchez et al., 1996). DiVerences in response of autistic symptoms to serotonergic drugs between autistic adults and children would be expected in light of the Wndings with [11C]-AMT PET that young autistic children have lower serotonin synthesis than normal children, whereas autistic men have higher serotonin synthesis than normal men.
Intriguingly, manipulations of serotonin in animals during preand postnatal development can reproduce some of the pathologic Wndings in autistic brain reported by Bauman and colleagues (Bauman and Kemper, 1985, 1994). For example, Bauman and Kemper (1985) reported reduced neuronal cell size and increased cell number in the hippocampus of autistic brains. Treatment of pregnant rats with p-chlorophenylalanine to deplete serotonin resulted in a prolongation of the period of cell division in the pups in brain regions with dense serotonergic innervation, leading to increased neuronal cell numbers in hippocampus, superior colliculus, and several thalamic nuclei (Lauder and Krebs, 1978). Bauman and Kemper (1994) reported decreased complexity and extent of dendritic
arbors in the hippocampus of autistic brains. In the animal literature, Yan et al. (1997) have reported that depletion of serotonin with p-chlorophenylalanine or 5,7-dihydroxy- tryptamine in neonatal rat pups resulted in large decreases in the numbers of dendritic spines in the hippocampus.
The focal decreases in [11C]-AMT that were observed in the cortex and thalamus might be interpreted in light of developmental changes in serotonin mechanisms shown in animal studies. For example, it has been demonstrated recently that the serotonin transporter is transiently expressed by glutamatergic thalamocortical aVerents (Lebrand et al., 1996; Bennett-Clarke et al., 1996) during the Wrst 2 postnatal weeks in rats (note that a serotonin transporter polymorphism that is not eYciently transcribed shows a high incidence in the autistic individuals and their families (Cook et al., 1997)). During this period, these thalamocortical neurons take up and store serotonin, although they do not synthesize serotonin. While the role of serotonin in glutamatergic neurons with cell bodies located in the sensory nuclei of the thalamus is not yet known, there is evidence that the serotonin concentration must be neither too high nor too low during this period. Depletion of serotonin delays the development of the barrel Welds of the rat somatosensory cortex (Blue et al., 1991; Osterheld-Haas and Hornung, 1996) and decreases the tangential arborization of the thalamocortical axons, resulting in reduced size of the barrel Welds (BennettClarke et al., 1994). Conversely, increased serotonin during this critical period results in increased tangential arborization of these axons and blurring of the boundaries of the cortical barrels (Cases et al., 1995, 1996). Developmental changes in serotonergic mechanisms have also been reported in the cerebellum. The 5HT1A receptors are transiently expressed in cerebellum during brain development. In rat pups, there is high expression of the 5HT1A receptor in the Purkinje cell layer between postnatal days 2 and 9, but there is no detectable 5HT1A receptor in the cerebellum of adults (Miquel et al., 1994). These data suggest an important role for serotonin in Purkinje cells of the cerebellum during brain development. Bauman and her colleagues have also reported decreased numbers of Purkinje cells in the cerebellum (Bauman and Kemper, 1985) in autistic brains. These Wndings are particularly interesting in light of the focal increase in [11C]-AMT demonstrated in the dentate nucleus of the cerebellum (Chugani et al., 1997), since Purkinje cells project to the dentate nucleus. In sum, the frontal cortex, thalamus, and cerebellum may be particularly sensitive to changes in serotonin synthesis during development, and the abnormalities in these regions measured with [11C]-AMT PET may stem from develop-
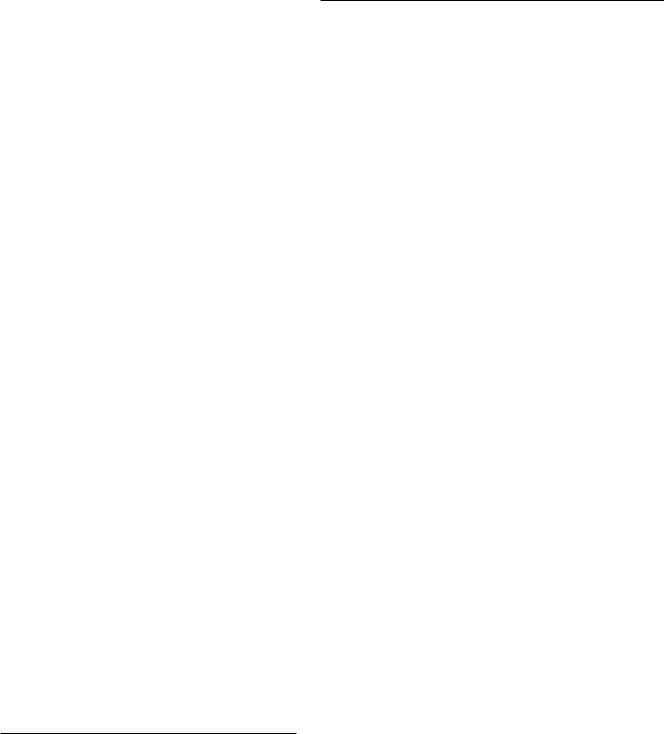
Autism 181
Fig. 10.2. Absolute values of local cerebral metabolic rates for glucose for cerebral cortex plotted as a function of age in 29 infants and children and seven young adults. In infants and children, points represent individual values; in adults, points are mean values from seven subjects, in which the size of the symbols equals the standard error of the mean. (Courtesy of Harry T. Chugani.)
mental alterations in serotonin synthesis. Focal alterations in [11C]-AMT accumulation may represent either aberrant innervation by serotonergic terminals or altered function in anatomically normal pathways. Immunocytochemical studies in pathologic specimens are necessary in order to determine which is the case. Altered serotonergic neuromodulation of dentatothalamocortical synaptic activity could be one pathophysiologic mechanism underlying sensory dysfunction in autism.
Problems that need to be addressed in future studies of autism
Brain functional changes with development
Since autism is most commonly recognized in the second year of life, associated with regression in both social behavior and language in up to half of autistic children (Tuchman and Rapin, 1997), the study of young children soon after identiWcation is more likely to yield important
information regarding the etiology of the disorder than the study of older autistic individuals, who are likely to have undergone various interventions. Furthermore, although autism is not a progressive disorder in the classical sense, the behavioral manifestations of autism do change with age, and, therefore, functional brain abnormalities also might change with age. Since functional imaging has demonstrated striking changes in brain glucose metabolism during the normal maturational process (Chugani et al., 1987), an evolution of brain regional values for other markers of brain function in autistic children compared with age-matched normal children may occur during development.
Quantitative analysis of local CMRGlu (lCMRGlu) shows that the brain follows a protracted glucose metabolic maturational course (Chugani et al., 1987). Neonatal lCMRGlu values, which are about 30% lower than adult rates, rapidly increase to exceed adult values by 2±3 years of age in the cerebral cortex and remain at these high levels until about 8±10 years, when lCMRGlu declines to reach adult rates by 16±18 years (Fig. 10.2). Correlation of these CMRGlu

182D. C. Chugani
trends with other neurodevelopment events suggests that the ascending portion of rapid lCMRGlu increase corresponds to the period of rapid overproduction of synapses and nerve terminals known to occur in the human brain (Huttenlocher, 1979; 1990; Huttenlocher et al., 1982; Huttenlocher and de Courten, 1987). The ªplateauº period, during which lCMRGlu exceeds adult values, corresponds to the period of increased cerebral energy demand as a result of transient exuberant connectivity relative to synaptic elimination. The segment of the metabolic maturational curve that corresponds to the lCMRGlu decline (between 10 and 16 years) corresponds to the period of selective elimination or ªpruningº of excessive connectivity.
Developmental studies in nonhuman primates also demonstrate nonlinear developmental changes in neurotransmitter content and receptor binding (GoldmanRakic and Brown, 1982; Lidow et al., 1991). For example, in the macaque, there is a steep rise in serotonin content in cortex beginning before birth and reaching a peak at 2 months of age, followed by a slow decline up to 3 years of age, when puberty occurs (Goldman-Rakic and Brown, 1982). The same group of investigators has reported a similar time course for expression of serotonin receptors (Lidow et al., 1991). These changes in serotonin markers parallel changes in synaptic number in the macaque cortex (Rakic et al., 1986; Bourgeois et al., 1994). Furthermore, these changes in synaptic number show a similar time course to that of the developmental changes in glucose metabolism in macaque and vervet monkeys (Jacobs et al., 1995).
In sum, it appears essential to include young children in functional imaging studies of autism to determine whether there are metabolic or neurochemical alterations during the process of brain development. The identiWcation of speciWc neurochemical abnormalities during postnatal brain development in autism might lead to new strategies for intervention. Because of the dynamic nature of developing brain function, imaging studies in children need to take into account relatively large changes with age. While the use of age-matched control subjects will guard against drawing invalid conclusions that are artifacts of age diVerences between groups, this approach may decrease the sensitivity in detecting diVerences between groups owing to the introduction of age-generated variability. Muzik et al. (1999) have attempted to address this problem by devising a mathematical developmental function with identiWable parameters representing diVerent stages of development, such as the CMRGlu value of the ªplateau phaseº and the age at which it begins to decline, that can be statistically compared among groups.
Control subjects or comparison groups
Normal controls
The most overwhelming barrier to functional imaging of disorders of childhood is obtaining an appropriate agematched normal control group. The risks of performing functional imaging studies with PET or SPECT include exposure to ionizing radiation and sedation. Ernst et al. (1998) reviewed studies of low-level radiation exposure with large sample sizes and long follow-up and concluded that health risks from low-level radiation could not be detected above those of adverse events of daily life. Furthermore, they found no evidence that low levels of radiation were more harmful to children than to adults. Nonetheless, current recommendations for radiation dosimetry in children suggest a 10-fold lower dose limit for minors (less than 18 years of age) compared with adult doses (IRCP, 1988). Consequently, while the risks of most functional imaging studies to older children are acceptable, control studies of normal children less than the age of 6±8 years are more problematic. First, they are more diYcult because of the need for cooperation and, in particular, the need to stay still in a scanner for long periods of time (for example, 30min for an FDG scan and 90min for a [11C]-AMT scan). Because of this requirement, sedation must be employed for many imaging procedures, adding to the overall risk. However, several large studies indicate that this is a small risk, and that sedation of children can be done in a safe and highly eYcacious manner in a hospital radiology department using a structured sedation program modeled after the guidelines of the American Academy of Pediatrics (Merola et al., 1995; EgelhoV et al., 1997). Second, very young children are not as capable of coping with the insertion of intravenous needles and the presence of a laboratory environment. Behavioral methods have been developed to address these issues for children. Acclimation techniques and strategies such as making the scanning environment more child-friendly (for example, using a facade to make the scanner look like a spaceship) have resulted in decreased stress to the children and increased cooperation, diminishing the need for sedation (Slifer et al., 1993, 1994; Rosenberg et al., 1997).
Siblings as a comparison group and the expanded phenotype of autism
Ethical guidelines recommend the study of children for comparison groups who may derive direct beneWt from the study. Since there is considerable evidence that autism is genetically transmitted through family members carrying a milder phenotype (see below), the normal siblings of autistic children have served as control subjects. Older
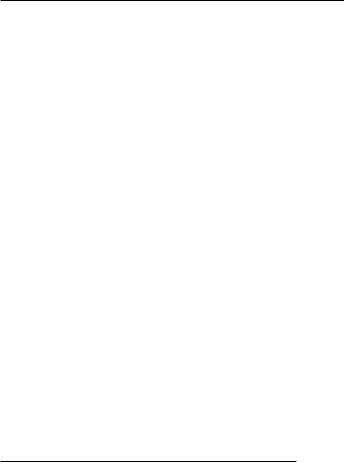
Autism 183
children with an autistic sibling are capable of understanding the psychologic, social, and economic impact of this genetic disorder on their family, as well as the value of their participation in the study. As a precaution against parental coercion, the child should be interviewed in the absence of the parents to ascertain the child's motivation (or lack of motivation) for participation in the study before obtaining the child's written assent.
Family and twin studies (reviewed in Piven and Folstein, 1994; Ciaranello and Ciaranello, 1995) have provided compelling evidence that genetic factors are important in the etiology of autism. The frequency of autism in subsequently born siblings is estimated at 6±8%, which is up to 200 times the risk in the general population (Bailey et al., 1996). Furthermore, three twin studies have detected pairwise concordance rates of approximately 65% in monozygotic and 0% in dizygotic pairs, producing a heritability estimate of over 90% (Folstein and Rutter, 1977; SteVenburg et al., 1989; Bailey et al., 1995). Epidemiologic, twin, and family studies have suggested that familial transmission of autism may involve a milder form of the disorder with an expanded autistic phenotype (Folstein and Rutter, 1988; Folstein and Piven, 1991; Landa et al., 1992). This phenotype is characterized by certain social personality characteristics, pragmatic language deWcits, and a rigid style of behavior that are qualitatively similar to, but milder than, those features which deWne autism (Piven et al., 1994, 1997; Santangelo and Folstein, 1995). For example, Piven et al. (1994) found that parents and adult siblings often rated themselves as socially aloof, undemonstrative of emotion, unresponsive to the emotional cues of others, and untactful. Landa et al. (1990, 1991) detected marked deWcits in the social use of language in the parents of autistic individuals compared with controls. Parents of autistic children were judged to provide too much detail and to give vague accounts, to be unable to give up their conversational turn, and to misinterpret statements frequently. High rates of anxiety and aVective disorders have been reported in the family members of autistic individuals (reviewed in Piven and Folstein, 1994). Le Couteur et al. (1996) studied the behavioral phenotype in 28 monozygotic pairs and 20 dizygotic same-sex twin pairs in which one or both twins had autism. In the nonautistic twins, there was a high incidence of language delay and social deWcits. Concordance for this broader phenotype was much greater in monozygotic than dizygotic twins, indicating a strong genetic component. Functional brain abnormalities underlying these behavioral deviances are also likely to be manifested in the siblings with the broader phenotype. However, the magnitude of these abnormalities are likely to be smaller,
such that signiWcant diVerences between autistic children and their siblings with a milder phenotype will be detectable.
Disease controls
Another approach for obtaining a comparison group is to employ a group of children with a diVerent disorder but with otherwise normal development who might beneWt from the study. This type of control does not constitute an ideal comparison group since their imaging studies will show abnormalities related to their disorder. If the abnormalities are limited to a certain brain region, the remainder of the brain might be used for comparison to the autistic group. Children with epilepsy, for example, have been shown to have focal increases in the uptake of the tracer [11C]-AMT associated with epileptogenic cortex. Values for whole brain serotonin synthesis capacity have been calculated for children with epilepsy excluding the epileptogenic region for comparison of whole brain values in autistic children (Chugani et al., 1999a). It must be kept in mind when using this experimental approach, however, that adaptive changes can take place in other areas of the brain in response to the initial lesion.
Conclusions and future directions
Studies of glucose metabolism and blood Xow have suggested a variety of global and focal brain abnormalities in autism. Newer more speciWc neurotransmitter probes, functional mapping, and MRS have begun to provide new clues to the biology of autism. However, data from the various imaging modalities have not yet converged to provide a unifying hypothesis of brain mechanisms. Brain regions implicated by functional imaging studies of autistic subjects are illustrated in part in Fig. 10.3. Frontal, medial prefrontal, temporal, and anterior cingulate cortical regions have been implicated with the various tracers. Abnormalities have also been reported in subcortical regions, such as basal ganglia, thalamus, and, cerebellum.
It is clear that functional imaging studies in young children, as well as new strategies for the assessment of developmental functional imaging data, are necessary to advance our understanding of autism. Furthermore, attention to the details of the design of future studies is essential. It is suggested that samples should not be merely gender matched, but that data from males and females should be analyzed separately because of gender diVerences in metabolic rates (Baxter et al., 1987), neurotransmitter synthesis (serotonergic gender diVerences reviewed in Chugani et al., 1998), and age of onset of