
Advanced Wireless Networks - 4G Technologies
.pdf
MULTITIER WIRELESS CELLULAR NETWORKS |
679 |
the cell radius can shrink to as small as 50 m (compared with 0.5–10 mile radius range for today’s macrocellular systems). Smaller cell radii are also possible for systems with smaller coverage, such as picoand nanocells in a local-area environment. The size of the cells is closely related to the expected speed of the mobiles that the system is to support. In other words the faster the mobiles move, the larger the cells should be. This will keep the complexity of handoffs at a manageable level. The cell size is also dependent on the expected system load (Erlangs per area unit). The larger the system load, the smaller the cell should be. This is the direct outcome of the limited wireless resources assigned to handle cell traffic. Smaller cells result in channels being reused a greater number of times and thus greater total system capacity. Finally, the cell size also depends on the required QoS level. This includes the probability of call blocking, call dropping or call completion. In general, the more stringent the QoS, the smaller the cells need to be, since smaller cells increase the total system capacity. Unfortunately, smaller cells require more base stations, and hence higher costs.
In this section, we discuss the multitier concept to show how to optimize the design of a system with differing traffic requirements and mobile characteristics. We examine how to lay out a multitier cellular system, given a total number of channels, the area to be covered, the average speed of mobiles in a tier, call arrival and duration statistics for each tier, and a constraint on the QoS (i.e. blocking and dropping probabilities). We discuss how to design a multitier cellular system in terms of the number of tier-i cells (e.g. of macrocells and microcells in a two-tier system) and the number of channels allocated to each tier so that the total system cost is minimized.
17.3.1 The network model
For each tier, the total area of the system (coverage area) is partitioned into cells. All cells of the same tier are of equal size. The network resources are also partitioned among the network tiers. Channels allocated to a particular tier are then reused based on the reuse factor determined for the mobiles of that tier (i.e. within each tier, channels are divided into channel sets). One such set is then allocated to each cell of that tier. For simplicity, FCA for the allocation of channels both among the tiers and within a tier is used.
In this section, connection-oriented traffic is considered. Each tier is identified by several parameters: call arrival rate, call duration time, call data rate, average speed of mobiles, performance factors and QoS factors. Additional assumptions are that:
handoffs and new calls are served from the same pool of available channels which means that, for a given tier, the probability of blocking equals the probability of dropping;
the speed of each class of mobiles is constant for each tier;
new call arrivals and call terminations are independent of the handoff traffic;
the traffic generation is spatially uniformly distributed;
call arrivals follow a Poisson process;
the spatial and time distribution of call arrivals are the same over all cells in a given tier, but can be different from tier to tier;
call duration for a given tier is exponentially distributed, and may vary from tier to tier.

MULTITIER WIRELESS CELLULAR NETWORKS |
681 |
||||
|
2 |
2 |
2 |
|
|
2 |
1 |
1 |
|
2 |
|
2 |
1 |
0 |
1 |
2 |
|
2 |
1 |
1 |
|
2 |
|
|
2 |
2 |
2 |
|
|
Figure 17.11 Cell splitting in multitier network.
structured splitting cells of tier i form rings within a cell of tier (i − 1) as shown for the two-tier case in Figure 17.11. Thus, if a base station already exists in a cell for tier i, the cost of placing an additional base station for a higher tier is negligible. Therefore, for example, in the case of a two-tier network the total cost can be reduced by the cost of the tier 2 base stations. In such a case, the total cost is
S |
i |
i−1 |
S |
i−1 |
TSC = |
γi |
m j − m j = γ1m1 + |
γi (mi − 1) |
m j , S ≥ 1 (17.18) |
i=1 |
j=1 |
j=1 |
i=2 |
j=1 |
It will be further assumed that the set of all the available channels to tier i, Ci , is equally divided among the fi cells in the frequency reuse cluster, which means no channel sharing between the cells. There is no channel sharing among the tiers either. It is also assumed that no channels are put aside for handling handoffs. Therefore, the blocking and dropping probabilities are equal, and will be referred to as the probability of loss, PL(i); that is,
i, PL(i) = PB(i) = PD(i) and PLmax(i) = min PBmax(i), PDmax(i)
The overall system loss probability, PLT, is given by:
S
|
|
PL(i)λ0 |
|
|
|
i=1 |
i |
|
|
PLT = |
S |
(17.19) |
||
|
||||
|
|
λ0 |
|
|
|
|
i |
|
i=1
The layout design problem is to minimize TSC, when the total number of available channels
is at most C, and subject to the following QoS |
constraints: PLT |
≤ |
PLT |
¯ |
¯ max |
|||||
|
S |
|
max and PL |
≤ PL ¯ . In |
||||||
other words: Find {mi } minimizing TSC with |
j=1 C j ≤ C and PLT ≤ PLTmax and PL ≤ |
|||||||||
¯ max |
[21]. |
|
|
|
|
|
|
|
|
|
PL |
|
|
|
|
|
|
|
|
|
|
|
The average number of handoffs, hi , that a call of tier i will undergo during its |
|||||||||
lifetime is |
|
|
|
|
|
|
||||
|
|
√ |
|
|
|
i = 1, 2, . . . , S [22] |
|
|||
|
|
hi = (3 + 2 3)Vi /9μi Ri , |
|
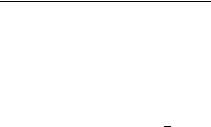
682 |
NETWORK DEPLOYMENT |
|
Now assume that there are a total of Mi cells in tier-i (Mi = |
i |
|
j=1 m j ). Then the total |
average number of handoffs per second in all the cells is hi λi Mi . The total arrival rate of
tier i cells is λ |
(hi + 1), and the average total (handoffs and |
||||||||||
handoff and initial calls in alltotal |
|
|
i Mi |
||||||||
initial calls) rate per cell is λ |
|
|
|
|
|
assumed that the total arrival process |
|||||
i |
|
= λi (hi + 1). It was total |
. Since the area of a hexagon with |
||||||||
to a cell is still Markovian, with the average rate of λi |
|
||||||||||
radius R is 3√3/2R2, we obtain λ |
= |
3√2λ0 R2/2. This leads to |
|||||||||
|
|
i |
i |
i |
|
|
|
|
|
||
|
|
|
|
√ |
|
|
√ |
|
|
|
|
total |
|
0 |
|
(2 + |
3)Vi + 3 |
|
|
3 |
μi Ri |
||
λi |
= λi |
Ri |
|
|
|
|
|
(17.20) |
|||
|
|
2μi |
|
|
The average call termination rate is [22] μitotal = μi (1 + 9hi ). We can use the Erlang-B formula (see Chapter 6) extended to M/G/c/c systems. The probability of loss of a tier i call is therefore
|
λtotal/μtotal |
Ni /Ni ! |
|
|
|
||
PL(i) = |
i |
i |
|
|
|
|
(17.21) |
Ci |
λtotal/μtotal j /j! |
|
|
|
|||
|
j=0 |
i |
i |
|
|
|
|
= |
{[λi (1 + hi )]/[μi (1 + 9hi )]} Ni /Ni ! |
, i |
= |
1, 2, . . . , S |
|||
Ci |
|
|
j |
|
|
||
|
j=0 |
{[(λi (1 + hi )]/[μi (1 + 9hi )]} /j! |
|
|
|
where λitotal, μitotal, λi and hi are given above, and Ni = Ci / f , i = 1, 2, . . . , S.
For a two-tier system (S = 2), the cost function simplifies to TSC = λ1 · m1 + λ2 · m1 · (m2 − 1). Parameters m1, m2 and R2 as a function of the area, A and the tier 1 cell radius,
R1, can be expressed as |
|
m1 = A/ A1 = 2√3A/9R12 |
(17.22) |
where A1 is area of tier 1 cell. We assume that both tier 1 and tier 2 cells are hexagonally shaped and that tier 2 cells are obtained by suitably splitting the tier 1 cells. Each tier 1 cell will contain k layers of tier 2 cells. Figure 17.11 shows an example of a tier 1 cell which contains three layers (0, 1 and 2). From the geometry of a hexagon, the number of tier 2 cells in a tier 1 cell is given by m2 = 1 + 6 + · · · + 6k = 1 + 3k(k + 1), k = 0, 1, . . . , where (k + 1) denotes the number of ‘circular layers’ in the cell splitting. Also, the radius of a tier-2 cell is given by R2 = R1/√m2.
The constrained optimization problem outlined above consists of finding optimal values of R1, R2, C1, C2, m1 and m2, which are the system design parameters. The optimal values will be referred to as R1 , R2 , C1 , C2 , m1 and m2. The parameters m1, m2, C1 and C2 are integers, while R1 and R2 are continuous variables. However, we only need to consider a discrete subset of the possible values of R1. From Equation (17.22), it is sufficient to consider just those values of R1 for which R1 = √(2√3A/9 ) where = mmin1, . . . , mmax1; mmin1 and mmax1 are the lower and upper bounds, respectively, of the number of tier 1 cells in the system. Thus, R1 can only assume one of mmax1 – mmin1 + 1 values.
The lower bound, mmin 1, is usually assumed to be equal to 1, while the upper bound, mmax 1, can be estimated from TSCmax, which is given to the designer, as mmax 1 =TSCmax/γ1 . The following search procedure is used in Ganz et al. [21] to find the design

MULTITIER WIRELESS CELLULAR NETWORKS |
683 |
parameters.
TSC* = TSCmax
mmax 1 = TSCmax/γ1
√
a1 = 3 2λ01/2
√
a2 = 3 2λ02/2
√
b1 = (3 + 2 3)V1/9μ1
√
b2 = (3 + 2 3)V2/9μ2
√
c = 2 3A/9
while (mmin 1 ≤ m1 ≤ mmax 1) do
R1 = c/√m1
λ1 = a1 R12
h1 = b1/ R1
while (1 ≤ C1 < C) do
N1 |
= C1/ f1 |
|
|
|
|
|
||||
N2 = (C − C1) / f2 |
|
|
|
|
|
|||||
P (1) |
= |
{[λ1(1 + h1)]/[μ1(1 + 9h1)]}N1 /N1! |
|
|||||||
L |
Ni |
(1 |
+ 9h1)]} |
j |
/j! |
|||||
|
|
j=0 {[λ1(1 + h1)]/[μ1 |
|
|||||||
for (k = 1;; k++) do |
|
|
|
|
|
|
|
|
|
|
|
|
m2 = 1 + 3k(k + 1) |
|
|
|
|
|
|||
|
|
TSC = γ1m1 + γ2m1(m2 − 1) |
|
|
|
|||||
if (TSC > TSC*) then break |
|
|
|
|
|
|||||
R2 = R1/√ |
|
|
|
|
|
|
|
|
||
m2 |
|
|
|
|
|
|||||
λ2 |
= a2 R22 |
|
|
|
|
|
||||
h2 |
= b2/ R2 |
|
|
|
|
|
||||
P (2) |
= |
{[λ2(1 + h2)]/[μ2(1 + 9h2)]}N2 /N2! |
|
|||||||
|
|
|||||||||
L |
N2 |
(1 |
+ 9h2)]} |
j |
/j! |
|||||
|
|
j=0 {[λ2(1 + h2)]/[μ2 |
|
|||||||
PLT |
= |
λ10 PL (1) + λ20 PL (2) |
|
|
|
|
|
|
||
|
|
|
|
|
|
|||||
|
|
λ10 + λ20 |
|
|
|
|
|
if (PLT < PLTmax) and [PL (1) < PLmax(1)] and [PL (2) < PLmax(1)] then break end for

684 NETWORK DEPLOYMENT
if (TSC < TSC*) then
ˆ = k k
m1 = m1 m2 = m2 R1 = R1
R2 = R1/√m2 C1 = C1
C2 = C − C1
end if end while
end while
The outputs are: TSC , m1, m2, R1 , R2 , C1 and C2 .
17.3.2 Performance example
To generate numerical results, a system with the parameters shown in Table 17.1 is used [21]. The performance of the two-tier system is compared with that of a one-tier system. To obtain results for a one-tier system the optimization algorithm with R1 = R2 was run. This results in both tiers sharing the same cells and m2 = 1. The total cost is then computed as TSC = m1γ 1.
Table 17.1 Example system parameters
Parameter |
Value/range |
Units |
|
|
|
A |
100 |
km2 |
C |
90 |
Channels |
S |
2 |
|
λ0 |
0.23.0 |
Calls/(min km2) |
1 |
|
|
λ0 |
5.040.0 |
Calls/(min km2) |
2 |
|
|
μ1, μ2 |
0.33 |
Calls/min |
γ 1 |
10.0 |
$ (in 1000 s)/base |
γ 2 |
1.0 |
$ (in 1000 s)/base |
V1 |
30540 |
km/h |
V2 |
1.512.0 |
km/h |
f1, f2 |
3 |
|
TSCmax |
10 000–20 000 |
$ (in 1000 s) |
PLTmax |
0.01 |
|
Pmax(*) |
0.01 |
|
B |
|
|
Pmax(*) |
0.01 |
|
D |
|
|
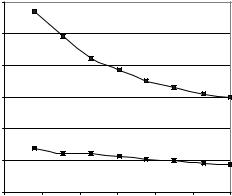
LOCAL MULTIPOINT DISTRIBUTION SERVICE |
685 |
|
1200 |
|
|
|
|
|
|
s) |
1000 |
|
|
|
|
|
|
($1000 |
|
|
|
|
|
|
|
|
|
|
One-tier system |
|
|
||
800 |
|
|
|
|
|
|
|
cost |
|
|
|
|
|
|
|
|
|
|
|
|
|
|
|
system |
600 |
|
|
|
|
|
|
400 |
|
|
|
|
|
|
|
Total |
|
|
|
|
|
|
|
|
|
Two-tier system |
|
|
|
||
200 |
|
|
|
|
|
|
|
|
|
|
|
|
|
|
|
|
0 |
|
4 |
|
|
10 |
12 |
|
0 |
2 |
6 |
8 |
Average tier 2 mobile speed (km/h)
Figure 17.12 Comparison of the total system costs between the one-tier and two-tier systems, λ01 = 1[calls/(min km2)] and λ02 = 20[calls/(min km2).]
Figure 17.12 depicts the total system cost as a function of tier 2 mobile speed, while tier 1 mobile speed is fixed for one-tier and a two-tier systems. The tier 1 mobile speeds considered are 30, 90, 180, 270, 360 and 540 km/h.
The main conclusion from Figure 17.12 and many other similar runs [21] is that, for the parameter ranges used in this study, the two-tier system outperforms the single-tier system for all the values of the slower and faster mobile speeds.
17.4 LOCAL MULTIPOINT DISTRIBUTION SERVICE
Wireless systems can establish area-wide coverage with the deployment of a single base station. The local multipoint distribution service (LMDS) offers a wireless method of access to broadband interactive services. The system architecture is considered point-to-multipoint since a centralized hub, or base station, simultaneously communicates with many fixed subscribers in the vicinity of the hub. Multiple hubs are required to provide coverage over areas larger than a single cell. Because of the fragile propagation environment at 28 GHz, LMDS systems have small cells with a coverage radius on the order of a few kilometers. Digital LMDS systems can flexibly allocate bandwidth across a wide range of bi-directional broadband services including telephony and high-speed data access.
Multiple LMDS hubs are arranged in a cellular fashion to reuse the frequency spectrum many times in the service area. Complete frequency reuse in each cell of the system is attempted with alternating polarization in either adjacent cells or adjacent hub antenna sectors within the same cell. Subscriber antennas are highly directional with roughly a 9 inch diameter (30–35 dBi) to provide additional isolation from transmissions in adjacent cells and to reduce the received amount of multipath propagation that may cause signal degradation. Since cells are small and the entire spectrum is reused many times, the overall system capacity is quite high, and backhaul requirements can be large. Backhaul networks will probably be a combination of fiber-optics and point-to-point radio links.
686 NETWORK DEPLOYMENT
The system capacity comes mainly from the huge radio frequency (RF) bandwidth available: block A, 1150 MHz (27.50–28.35, 29.100–29.250 and 31.075–31.225 GHz); and block B, 150 MHz (31.000–31.075 and 31.225–31.300 GHz). For the purpose of frequency planning for two-way usage it is essential to solve the problem of LMDS spectrum partitioning for the upstream and downstream. The standard duplexing options, frequency-division duplex (FDD) and time-division duplex (TDD), are applicable in LMDS too and will not be discussed here in any more detail. Instead, as a wireless point-to-multipoint system, the deployment of LMDS will be assessed by the basic parameters of cell size and capacity. Obviously, an operator would want to cover as large an area as possible with a minimum number of cell sites, hence maximizing cell size. The modulation and coding, and the ensuing Eb/N0, are factors in determining cell size. However, because of high rain attenuation at LMDS frequencies, there is a major trade-off between cell size and system availability determined by the rain expectancy for a given geographical area. In most of the United States, for the forthright aim of providing ‘wireless fiber’ availability of 0.9999–0.99999, the cells would only be between 0.3 and 2 miles [23]. Consequently, the deployment of LMDS will extensively involve multicell scenarios. In the following we review the methods of optimizing system capacity in these scenarios through frequency reuse. The main problem related to frequency reuse is the interference between different segments of the system. Therefore, patterns that create bound and predictable values of S/I are essential to device deployment. Frequency reuse and capacity in interference-limited systems have been discussed in previous sections for mobile cellular and personal communications services (PCS) systems. As the basic methods and principles also apply to fixed systems, there are several major differences in the treatment of fixed broadband wireless systems such as LMDS.
Unlike mobile cellular, in LMDS the subscriber antennae are highly directional and point toward one specific base station. This, together with the nonmoving nature of the subscriber, results in much lower link dynamics. The channel can mostly be described as Rician (with a strong main ray) and not Rayleigh-like in mobile cellular. Since the subscriber employs directive antennae, it is compelled to communicate with only one base station, which excludes the use of macro diversity (or cell diversity) – a very beneficial method with mobile cellular, but one that complicates the interference and frequency reuse analysis.
Also, mobile cellular service was originally intended for voice; therefore, it is designed for symmetric loading, while broadband wireless services generally have more downstream traffic than upstream. This reflects in the main issue of concern for interference study, which in PCS and mobile cellular is upstream interference because the base station receiver has the hard task of receiving from a multitude of mobiles transmitting through nondirectional antennae and suffering different fast fading.
In LMDS the upstream is usually lower-capacity and employs lower-order modulation, which offers better immunity. Also, the slower fading environment allows a closed-loop transmission power control system to operate relatively accurately. Consequently, upstream most subscribers transmit at a power lower than nominal, unlike the downstream transmission. Also, the narrow beam of their antennae is an interference-limiting factor. Therefore, downstream interference is the most problematic, as opposed to the mobile cellular case.
Another parallel with mobile cellular refers to cellular patterns. Owing to the mobile nature of its subscribers, a mobile cellular system has to provide service from the start to a
LOCAL MULTIPOINT DISTRIBUTION SERVICE |
687 |
whole area (town, region), with a large number of adjacent cells. The number of sectors is relatively low (2–4); otherwise, the mobile would go through frequent handoffs.
In LMDS, in the long run, owing to the small cell size, the ever increasing hunger for bandwidth and the availability of radio and networking technologies, deployment is also expected to be blanket coverage. However, the economics of LMDS do not require this from the beginning. Operators will probably first offer the service in clusters of business clients with high data bandwidth demands and with the financial readiness for the new service, and later will gradually expand the service to larger areas. Second, the sectorization will be in denser patterns determined by the increasing demand for bandwidth and not limited by the requirement for handoff. The narrower sectors employing higher antenna gain in the base station also provide for larger cell size.
Frequency reuse in one cell is illustrated in Figure 17.13 with three examples of frequency reuse by sectorization. The first step in frequency planning is to assume the division of the available spectrum into subbands, so adjacent sectors will operate on different subbands. Also, the sector structure has to be designed as a function of the capacity required and the S/I specification of the modem used. The need to divide into subbands is a function of base station antenna quality, specifically the steepness of the rolloff from the main lobe to sidelobes in the horizontal antenna pattern. If the antenna sidelobes roll off very steeply after the main lobe, it is possible to reuse the frequency every two sectors, resulting in patterns of the type A, B, A, B, . . . , as in Figure 17.13(b). In this context the reuse factor, FR, is the number of times the whole band is used in the cell, which for the simplified regular patterns is the number of sectors divided by the number of subbands; in this case FR = 3. In a more conservative deployment the frequency is reused only in back-to-back sectors as in Figure 17.13(a), which has six sectors with reuse pattern A, B, C, A, B, C. Figure 17.13(c) shows a higher-capacity cell where the pattern A, B, C, . . . , is repeated in 12 sectors, resulting in FR = 4. Obviously, to keep the equipment cost low, we want a low number of sectors; schemes where we divide the spectrum in as few subbands as possible (two is best).
With present antenna technology at 28 GHz it is possible to achieve sidelobes under −33 dB, but the radiation pattern of the deployed antenna is different. The sidelobes are significantly higher because of scattering effects such as diffraction, reflection or dispersion caused by foliage. The very narrow beamwidth of the subscriber antenna (which at 28 GHz can be reasonably made 3◦ or lower) helps limit the scattering effect. Obviously, the sum of such effects depends on the millimeter-wave effects in the specific buildings and terrain in the area, and have to be estimated through simulation and measurements for each particular case. Another uncertain factor in the estimation of S/I is the fading encountered by the direct signal. As a starting assessment, we shall consider the above-mentioned effects accountable for increasing the equivalent antenna sidelobe radiation to −25 dB.
Based on this, the following estimations of S/I are only orientative. For each particular deployment the worst case has to be estimated considering the particular conditions.
17.4.1 Interference estimations
To allow A, B, C, . . . , type sectorization, in Figure 17.13(a) and (c) the base station antenna sidelobe is α = −25 dB at an angle more than 2.5 B3dB from boresight, where B3dB is the −3dB beamwidth (the main lobe is between ±0.5 B3dB). In Figure 17.13(a) and (b),