
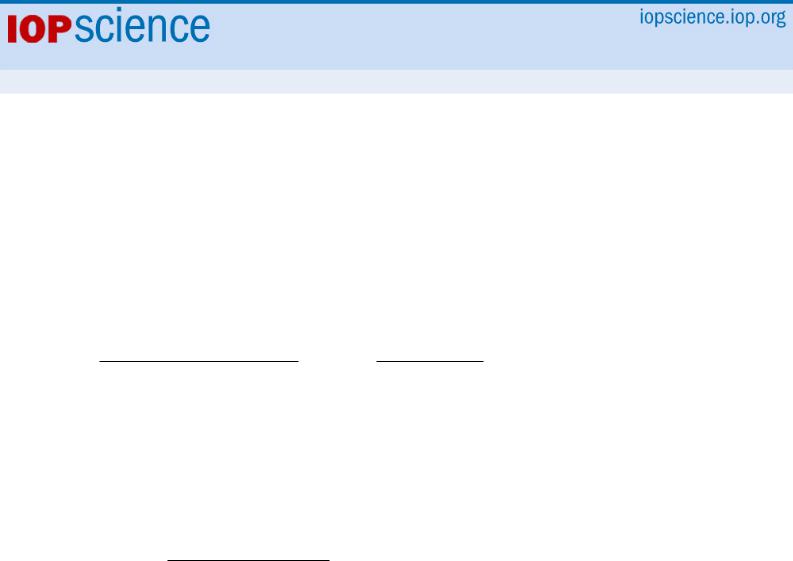
Home Search Collections Journals About Contact us My IOPscience
Structural and magnetic properties of Cr-doped Ni–Mn–In metamagnetic shape memory alloys
This article has been downloaded from IOPscience. Please scroll down to see the full text article.
2011 J. Phys. D: Appl. Phys. 44 395001
(http://iopscience.iop.org/0022-3727/44/39/395001)
View the table of contents for this issue, or go to the journal homepage for more
Download details:
IP Address: 212.193.134.82
The article was downloaded on 21/12/2011 at 06:20
Please note that terms and conditions apply.

IOP PUBLISHING |
JOURNAL OF PHYSICS D: APPLIED PHYSICS |
J. Phys. D: Appl. Phys. 44 (2011) 395001 (7pp) |
doi:10.1088/0022-3727/44/39/395001 |
Structural and magnetic properties of Cr-doped Ni–Mn–In metamagnetic shape memory alloys
V Sanchez´-Alarcos1, V Recarte1, J I Perez´-Landazabal´1, J R Chapelon1 and J A Rodr´ıguez-Velamazan´2,3
1Departamento de F´ısica, Universidad Publica´ de Navarra, Campus de Arrosad´ıa 31006 Pamplona, Spain
2Instituto de Ciencia de Materiales de Aragon,´ CSIC - Universidad de Zaragoza, Zaragoza, Spain
3Institut Laue-Langevin, CRG’s D1B D15, F-38042 Grenoble, France
E-mail: vicente.sanchez@unavarra.es
Received 25 March 2011, in final form 26 July 2011
Published 9 September 2011
Online at stacks.iop.org/JPhysD/44/395001
Abstract
The effect of the partial substitution of Mn by Cr on the structural and magnetic properties of Ni–Mn–In metamagnetic shape memory alloys is investigated. It is found that a Cr-rich second phase appears for quite low Cr concentrations, pointing out a very low solubility of Cr in Ni–Mn–In. Nevertheless, the martensitic transformation (MT) temperature of the doped alloys can be related to the variation in the electron concentration in the matrix phase, just as it occurs in the ternary Ni–Mn–In system. The effect of magnetic field on the structural transformation has been evaluated on both a ternary and a quaternary alloy. It is shown that the presence of the second phase reduces the magnetically induced shift of the MT and the associated magnetocaloric effect, thus limiting the potential applicability of Ni–Mn–In alloys. The obtained results prevent the addition of high amounts of Cr to Ni–Mn–In.
1. Introduction
Ferromagnetic shape memory alloys (FSMAs) have attracted considerable interest as a new type of materials for actuators and sensors due to the possibility of achieving giant magnetic field-induced strains. The peculiar properties of these alloys are linked to the occurrence of a thermoelastic martensitic transformation (MT); a solid–solid displacive, diffusionless, first-order transformation taking place from a high temperature phase (austenite) to a low-symmetry low-temperature phase showing different crystallographic structures depending on composition [1, 2]. In conventional FSMA, such as the prototypical Ni–Mn–Ga alloys, the MT takes place between ferromagnetic austenite and ferromagnetic martensite, in such a way that the ferromagnetic martensitic variants formed as a result of the MT may be rearranged by means of the application of an applied magnetic field, thus resulting in large magnetically induced strains [3, 4]. Nevertheless, a new type of alloys undergoing a MT from a ferromagnetic austenite to a very weak magnetic martensite, the so-called metamagnetic shape memory alloys, have recently drawn
more attention due to the potential of other interesting functional properties, such as the inverse magnetocaloric effect (MCE) [5–12] or the magnetoresitance [13–16], which are linked to a drastic magnetization change at the MT and, in particular, to the associated magnetic-field induction of the MT [17, 18]. The stress generated on the magnetic induction of the MT in metamagnetic alloys is about 50 times larger than the output stresses generated on the magnetically induced rearrangement of martensitic variants in conventional FSMA [17], so metamagnetic shape memory alloys turn out to be even more attractive than FSMA for practical applications such as magnetic actuation or sensing. Furthermore, the large MCE values obtained in these alloys also make them good candidates for magnetic refrigeration applications [19].
Metamagnetic behaviour has been reported in Ni–Mn–X (X = In, Sn, Sb and Al) alloys close to the stoichiometric composition N2MnX, which have been extensively studied over the past years. These are Heusler compounds showing
¯
a cubic L21 crystal structure (space group F m3m) and next- nearest-neighbours atomic ordering. The magnetism in these alloys is mainly due to the ferromagnetic coupling between the
0022-3727/11/395001+07$33.00 |
1 |
© 2011 IOP Publishing Ltd Printed in the UK & the USA |
J. Phys. D: Appl. Phys. 44 (2011) 395001 |
V Sanchez´-Alarcos et al |
Mn atoms located in the corresponding Mn sublattice, and, to a lesser extent, to the antiferromagnetic coupling between the Mn atoms in the correct sublattice and the Mn atoms in the X sublattice [20–22]. The compositional dependence of the MT temperature has been established in a broad compositional range and the crystal structure of the austenite and the different martensitic structures appearing in these alloys have been determined [23, 24]. The MT temperature has been proved to be very sensitive to composition, mainly through the valence electron concentration, e/a [24], so it can be properly tuned either by varying the chemical composition of the ternary alloy or by doping with an alloying element. In this sense, the partial substitution of Ni by Co has been shown to decrease the MT temperature and to increase the austenite Curie temperature of Ni–Mn–In, Ni–Mn–Sn, Ni–Mn–Sb and Ni–Mn–Al alloys, thus resulting in a considerable improvement of both the MCE and the magnetic induction of the MT [25–28]. In contrast, the MT temperature increases as a result of the partial substitution of Ni by Cu in Ni–Mn–In alloys [29]. It has also been shown that the partial substitution of Ni by Fe highly modifies the magnetocaloric properties of Ni–Mn–Sn [27, 30], leading to a decrease or to an increase in the MCE depending on the Fe content. The addition of Fe to the Ni–Mn–In system may also lead to an important improvement of the mechanical properties as a consequence of the appearance of a precipitated second phase [31]. On the other hand, giant low-field magnetic entropy changes and a considerable magnetically induced shift of the MT temperature have been reported as a result of the substitutions of Ni by Cu and of Mn by Cr, respectively, in Ni–Mn–Sn [32, 33]. Recently, it has been shown that partial substitution of small amounts (up to 2%) of Mn by Cr increases the MT temperature of Ni–Mn–In alloys, even though it means a decrease in the electron concentration in the alloy [34, 35]. This peculiar fact has been attributed to the effect of Cr on the ferromagnetic interactions in these alloys, in particular to a decrease in the ferromagnetic exchange (and therefore of the saturation magnetization) as a result of the partial substitution of Mn by Cr.
In this work, the effect of higher amounts of Cr on the Ni–Mn–In system is investigated. The microstructure of the quaternary Ni–Mn–In–Cr alloys has been analysed by scanning electron microscopy (SEM), and the structural and magnetic transformations have been studied by calorimetric and magnetization measurements. In particular, the effect of a magnetic field on the MT and the associated MCE effect have been studied in both the ternary and the Cr-doped alloys. It is found that a Cr-rich second phase appears for relatively low Cr concentrations, pointing out to the low solubility of Cr in the Ni–Mn–In system. Precisely, as a consequence of the appearance of the second phase, the addition of high amounts of chromium to Ni–Mn–In alloy is shown to be highly detrimental to the achievement of large MCE and large magnetically induced MT shifts, and therefore to the potential applicability of these alloys.
2. Experimental
Four polycrystalline ingots of nominal composition (at%) Ni50Mn33−x In17Crx (x = 0, 2, 3, 4) were prepared
from high purity elements by arc melting under protective Ar atmosphere. The ingots were remelted five times to ensure homogeneity and then subjected to a 1 h annealing treatment at 1073 K followed by quenching into ice water in a vertical furnace. Once treated, the composition of the elaborated alloys was analysed by EDS in a Jeol JSM5610LV Scanning Electron Microscope (SEM). Small samples for calorimetric and magnetic measurements were obtained from discs previously cut from the centre of the ingots by slow speed diamond saw. The transformation temperatures were determined from DSC measurements carried out at a cooling/heating rate of 10 K min−1 in a TA Q100 calorimeter. The magnetic characterization (magnetization measurements) was performed by SQUID magnetometry (QD MPMS XL-7 SQUID [36]).
3. Results and discussion
The microstructure of the elaborated alloys was studied by SEM. Figure 1 shows the backscattered-electron images obtained at room temperature. It can be seen that the microstructure considerably varies depending on the Cr concentration. In particular, the ternary alloy exhibits a singlephase microstructure whereas a second phase can be clearly distinguished in the Cr3 and Cr4 alloys and, to a lesser extent, in the Cr2 one. The fraction and the morphology of the appearing second phase drastically evolve with the increasing amount of Cr, from the few grain-boundaries located precipitates observed in the Cr2 micrograph to the cellular and dendritic structures shown in the Cr3 and Cr4 alloys, respectively. The chemical composition of the matrix and the second phase appearing in each alloy, as well as the average composition in the whole samples, are shown in table 1.
In all cases, the second phase is richer in chromium than the matrix phase, pointing out a low solubility of Cr in the Ni–Mn–In system. Up to now, the appearance of a second phase as a consequence of doping in Ni–Mn–In has just been reported from partial substitutions of more than 5% of Fe by In [31] or 7.5% of Co by Ni [26], so the observation of a precipitated phase in alloys with such a low Cr concentrations suggests that the solubility of Cr in Ni–Mn–In is the lowest among the studied doping elements. It is worth mentioning that no second phase has been reported in previous works on Cr-doped Ni–Mn–In alloys, although the Cr concentrations added to the ternary systems in those works were always below 2% (at%) [34, 35].
The structural transformations taking place in the studied alloys have been studied by DSC measurements performed on cooling–heating thermal cycles between 373 and 193 K. The DSC thermograms obtained on each alloy are shown in figure 2. The exothermic and endothermic peaks corresponding to the forward (austenite → martensite) and reverse (martensite → austenite) MTs can be clearly observed in the cooling and heating curves, respectively, where the transformation peaks show the typical thermal hysteresis inherent to its firstorder character. As a consequence of the extra energy term associated with the presence of the second phase, the transformation peaks are considerably broader for the Cr3 alloy
2

J. Phys. D: Appl. Phys. 44 (2011) 395001 |
V Sanchez´-Alarcos et al |
Figure 1. Room temperature SEM micrographs of the elaborated alloys: (a) Cr0, (b) Cr2, (c) Cr3 and (d) Cr4.
Table 1. Composition and valence electron concentration of the studied alloys.
Alloy |
Ni (at%) |
Mn (at%) |
In (at%) |
Cr (at%) |
e/a |
|
|
|
|
|
|
Cr0 |
50.3 |
32.5 |
17.2 |
0 |
7.821 |
Cr2 |
|
|
|
|
|
Average |
50.1 |
33.1 |
15.5 |
1.3 |
7.870 |
Matrix |
50.3 |
33.3 |
15.7 |
0.7 |
7.874 |
Precipitates |
45.6 |
32.3 |
14.8 |
7.3 |
7.703 |
Cr3 |
|
|
|
|
|
Average |
50.6 |
31.9 |
15.2 |
2.3 |
7.887 |
Matrix |
50.8 |
32.3 |
16.9 |
0 |
7.848 |
Precipitates |
54.9 |
31.1 |
10.3 |
3.7 |
8.198 |
Cr4 |
|
|
|
|
|
Average |
49.8 |
31.1 |
15.0 |
4.1 |
7.853 |
Matrix |
49.3 |
31.3 |
16 |
3.4 |
7.805 |
Precipitates |
51.5 |
29.5 |
11.4 |
7.6 |
8.013 |
|
|
|
|
|
|
and, especially, for the Cr4 one [37]. The MT temperature (MS) and the entropy change at the MT ( S) estimated from the thermograms are shown in table 2. MS has been taken as the onset on cooling of the forward MT peak (as indicated in the figure), and the entropy change has been calculated asS = H /T0, where H is the average enthalpy change between the forward and reverse MTs and T0 represents the mean MT temperature.
The magnetic transitions have been determined from magnetization measurements. Figure 3 shows the temperature dependence of the low-field magnetization on cooling and subsequent heating between 350 and 10 K at 100 Oe. The M(T ) curves are quite similar for the Cr0, Cr3 and Cr4 alloys. At high temperatures the samples are paramagnetic and they order ferromagnetically at the austenite Curie temperature TCaust. On cooling below MS, the magnetization drastically falls due to the transformation into paramagnetic martensite, which orders ferromagnetically on further cooling below the
martensitic Curie temperature, TCmart (as an example, the transformation temperatures corresponding the Cr0 alloy are indicated in the figure). This sequence of magnetostructural transitions is typically observed in Ni–Mn–In alloys and it is attributed to the weakening of the exchange interactions as a consequence of the abrupt change in the Mn–Mn interatomic distances occurring upon the MT [20]. If we compare figures 2 and 3, the fact that the peak observed on M(T ) around 280 K in the Cr3 alloy is considerably lower than that in the Cr0 and Cr4
alloys can be explained as a consequence of the smaller fraction of austenite present at TCaust in the Cr3 case. On the other hand,
the magnetization of the Cr2 alloy remains zero on cooling below MS, thus indicating that the MT takes place between paramagnetic phases (also compare figures 2 and 3). In this case, only the increase in magnetization associated with the ferromagnetic ordering of the martensite (at TCmart < MS) can be seen in the M(T ) curve. It is worth noting that no magnetic anomaly associated with the second phase is observed in the
3

J. Phys. D: Appl. Phys. 44 (2011) 395001 |
V Sanchez´-Alarcos et al |
Figure 2. DSC thermograms performed on a cooling/heating thermal cycle between 373 and 193 K.
Table 2. Transformation temperatures and entropy change at the MT.
Alloy |
MS (K) |
TCaust (K) |
TCmart (K) |
S (J kg−1 K−1) |
Cr0 |
288 |
288 |
211 |
36 |
Cr2 |
330 |
— |
142 |
44 |
Cr3 |
303 |
282 |
196 |
29 |
Cr4 |
289 |
285 |
230 |
28 |
|
|
|
|
|
Figure 3. Temperature dependence of the magnetization under a magnetic field of 100 Oe.
quaternary alloys. In fact, the absence of particular changes in the global magnetic behaviour under magnetic fields ranging from 100 to 60 kOe suggests a non-ferromagnetic nature of the second phase.
The structural and magnetic transformation temperatures, shown in table 2, show no obvious correlation with the Cr concentration, neither in the whole alloy nor in the matrix phase. This is not unexpected since the Mn concentration and the fraction of the second phase, which highly affects the net alloy magnetic moment and the transformation temperature
Figure 4. Structural and magnetic transformation temperatures as a function of the electron concentration, e/a, in the matrix phase.
range, respectively, are also different for the different alloys. Nevertheless, if the structural and magnetic transformation temperatures are plotted as a function of the matrix electron concentration (calculated as the sum of the 3d and 4s electrons of Ni, Mn and Cr, and the 5s and 5p electrons of In), as shown in figure 4, the obtained phase diagram is very similar to those in Ni–Mn–In alloys [21, 26, 38]. On the one hand, MS shows an almost linear increase with the increasing e/a ratio, as it does in Ni–Mn–In alloys, albeit the slope of the regression line is slightly lower in our quaternary system. On the other, TCaust is shown to be insensitive to the variation in composition whereas TCmart linearly decreases with the increasing e/a, just as it occurs in ternary alloys.
The fact that, irrespective of the Cr concentration, MS monotonically increases with the matrix electron concentration suggests that the addition of Cr affects the MT temperatures mainly through the variation in the e/a ratio. In this sense, the presence of the second phase seems not to affect the compositional dependence of the remanent matrix. In any case, more detailed compositional analysis should be performed to fully determine the phase diagram of Cr-doped Ni–Mn–In alloys.
In order to analyse the applicability of these alloys through the influence of a second phase, the magnetically induced shift of the MT and the associated MCE have been analysed and compared in the most extreme cases, that is, the ternary Cr0 alloy and the quaternary Cr4 alloy, the latter showing the larger fraction of second phase. It is worth noting that these two alloys show the same MS, which facilitates the analysis. The effect of magnetic field on the MT temperature has been evaluated from the analysis of temperature dependence of the magnetization on both alloys. Figure 5 shows the heating M(T ) curves obtained under different applied magnetic fields.
The shift of Tm is plotted in figure 6 as a function of the applied magnetic field. It can be seen that the MT temperature
of both alloys decreases with the increasing applied field, with slopes (dTm/dH ) = −0.09 K kOe−1 and (dTm/dH ) =
−0.09 K kOe−1 for the Cr0 and Cr4 alloys, respectively. This
4

J. Phys. D: Appl. Phys. 44 (2011) 395001 |
V Sanchez´-Alarcos et al |
Figure 5. Temperature dependence of the magnetization (heating curves) under different applied magnetic fields. (a) Cr0 and (b) Cr4 alloys.
magnetically induced shift of the MT can be evaluated on the basis of the Clausius–Clapeyron equation
dTm |
M |
(1) |
|
|
= −µ0 |
|
|
dH |
S |
where M is the difference in magnetization between the austenite and the martensite at the MT and S is the entropy change at the MT. In effect, if the magnetization shifts estimated from the M(T ) curves at 60 kOe ( M = 32 emu g−1 and M = 14 emu g−1, for Cr0 and Cr4 alloys, respectively) and the entropy change values obtained from DSC measurements (see table 2) are substituted in equation (1), the obtained shifts are (dTm/dH ) = −0.09 K kOe−1 for the Cr0 alloy and (dTm/dH ) = −0.05 K kOe−1 for the Cr4 alloy, which are in good agreement with the values obtained directly from the regression lines in figure 6. Nevertheless, these magnetically induced shifts of the MT are quite lower than those in similar Co-doped Ni–Mn–In alloys [39].
The low dTm/dH values obtained in the studied alloys are
explained as a consequence of the small differences between TCaust and MS, that is, the small (TCaust − MS) in both alloys, as
Figure 6. MT temperature shift on the Cr0 and Cr4 alloys as a function of the applied magnetic field.
long as it implies that M is still too small at the MT whereasS is close to its maximum value (since almost no magnetic contribution to the entropy change opposes to the vibrational one [40]), thus leading to really low values in the right-hand side of equation (1). Nevertheless, it is worth noting that, in spite of (TCaust − MS) being quite similar in the Cr0 and Cr4 alloys, dTm/dH is significantly lower in the Cr4 alloy, which shows indeed a lower S because of the presence of the nontransforming second phase. This is due to the fact that M in the ternary case is more than twice M in the quaternary alloy, which could be attributed to the above-mentioned reduction in the ferromagnetic exchange as a consequence of the partial substitution of Mn by Cr, as well as to the fact that, due to the great width of its MT temperature range, the magnetization of the martensitic phase appearing in the Cr4 alloy is considerably larger than in the Cr0 case (see figure 5). In any case, it can be concluded that the magnetically induced shift of the MT in Ni– Mn–In alloy, and therefore its potential applicability, clearly deteriorates on addition of 4% of Cr alloys.
The MCE has also been studied in the Cr0 and Cr4 alloys. The MCE is evaluated as the temperature change produced in a sample when a magnetic field is applied or removed under adiabatic condition, or, equivalently, as the entropy change under isothermal conditions. Both terms are related through the specific heat of the sample [41]. According to classical thermodynamics, the isothermal magnetic entropy change can be indirectly measured by numerical integration of ∂M/∂T from a set of magnetization versus temperature data at different values of applied field, following the expression
Sm = S(T , H ) − S(T , 0) = 0 |
H |
∂T |
H dH . (2) |
|
|
|
|
∂M |
|
In order to estimate the MCE, Sm has been calculated from the temperature dependence of magnetization on heating both alloys under applied magnetic fields ranging from 100 to 60 kOe. As expected, the highest values of Sm were obtained at the highest applied field. The calculated Sm under 60 kOe
5