
5 курс / Пульмонология и фтизиатрия / Clinical_Tuberculosis_Friedman_Lloyd_N_,_Dedicoat
.pdf
220 BCG and Other Vaccines
Table 12.1 Potential reasons for variability in BCG efficacy
Hypothesis |
Related literature |
Differences in BCG strains |
41,48,54,90–93 |
Exposure to NTM |
44,95–100,244 |
Blocking |
|
Masking |
|
Differences between host populations |
39,101–104,245 |
Genetic |
|
Nutritional |
|
Co-infections (viral, helminth) |
|
Environmental influences |
39,245 |
Sunlight exposure |
|
Variability in cold-chain maintenance
Differences in circulating M.tb strains
role in variable levels of efficacy.41 Furthermore, the same strain of BCG has been found to be effective in some populations but not in others.48,54 A critical review of animal and human studies found evidence to support the notion that protection afforded against TB differs between BCG-vaccine strains, but concluded that there are insufficient data to recommend one particular strain.93
EXPOSURE TO NON-TUBERCULOUS MYCOBACTERIA
Non-tuberculous mycobacteria (NTM) are saprophytic organisms which live in soil or water and do not cause disease except in immunosuppressed individuals. Exposure to both M.tb and NTM is greater in tropical regions, and several epidemiological observations have linked mycobacterial sensitization to the geographically associated reduction in BCG efficacy. BCG vaccination conferred effective protection in trials from which TST-positive (and therefore sensitized) donors were excluded,41,42 whereas in populations where BCG did not perform well, in Alabama and India, greater than 68% and 95% of individuals, respectively, were purified protein derivative positive by 15–20 years of age.44,94 In a comparative immunogenicity study, children from the UK had very low levels of baseline anti-mycobacterial immunity which were significantly increased following BCG vaccination, consistent with the known high efficacy of BCG in the UK.41 In contrast, in Malawi, where BCG does not protect, children had high baseline levels which were not boosted by BCG.95 A further study in Malawi found that individuals with lower immune responses to NTM showed greater interferon (IFN)-γ responses to BCG.96 The very cross-reactivity that allows BCG to impart protection against other mycobacteria such as M. leprae may be a double-edged sword.
Palmer and colleagues proposed that exposure to environmental mycobacteria offers some level of protective immunity to TB that BCG can do little to improve on. This “masking hypothesis” is supported by guinea-pig experiments in which animals immunized with various NTM demonstrated immunity to M.tb and a reduction in the protective efficacy of a subsequent BCG vaccination.97 Alternatively, according to the “blocking hypothesis,” pre-existing immunity to antigens common across mycobacterial species may block the ability of BCG to replicate and induce a protective immune response. In a murine study, prior exposure to NTM resulted in a broad immune response that was recalled rapidly after BCG
vaccination and restricted BCG multiplication, with no protective immunity mounted against M.tb; the efficacy of non-replicating subunit vaccines was unaffected.98 In human trials, accelerated waning of skin-test responses has been observed in Malawi and India, suggesting transient secondary responses recalled from sensitization to NTM or latent M.tb infection.44,99 Masking and blocking are not mutually exclusive and may work together to interfere with BCG efficacy in high NTM endemic areas.
Interestingly, there is also some evidence from murine experiments that exposure to NTM can induce opposite effects on BCG efficacy depending on the route of exposure and viability of NTM.100 Further studies applying reproducible preclinical models of NTM exposure and relating quantitative measures of NTM responses to BCG efficacy in humans are required to evaluate the role of anti-mycobacterial immunity in BCG interference. However, such assessment is hampered by the lack of sufficiently immunogenic NTM-specific antigens, with studies to date relying on the use of differential responses to purified protein derivatives from NTM and M.tb.95
OTHER FACTORS
The variability in BCG efficacy has also been attributed to genetic or nutritional differences between host populations, environmental influences such as sunlight exposure, variability in coldchain maintenance, and differences in circulating M.tb strains. However, there is no convincing evidence of a major role for these factors; in particular, the finding that BCG can protect against leprosy where it fails against TB throws into question many of these hypotheses.39
Recent evidence from a case–control correlates of risk study found that activated T-cells, driven in part by cytomegalovirus (CMV) infection, were associated with an increased risk of TB disease in BCG-vaccinated South African infants.101 It is possible that viral infections during the development of the BCGspecific immune response impair the development of protective immunity, as supported by previous studies in Malawi and the Gambia.102,103 Helminth infections, which are more prevalent in tropical and subtropical regions, may also reduce the immunogenicity of BCG.104
PROTECTIVE IMMUNITY AGAINST
MYCOBACTERIUM TUBERCULOSIS
Rational design of a more effective TB vaccination regimen relies upon an understanding of the immune mechanisms of protective immunity. In other diseases, such as meningococcal disease, the existence of a validated immunological correlate of protection greatly facilitates vaccine development.105 There is no clear correlate of protection for TB, but we do have some understanding of immune responses that are necessary and important.
Cellular immunity
Due to the intracellular nature of mycobacteria, cell-mediated immunity is central to protection against M.tb and control of infection. Mice deficient in CD4+ T-cells or major histocompatibility
Книга в списке рекомендаций к покупке и прочтению сайта https://meduniver.com/

New vaccine approaches 221
complex class II are unable to control bacterial growth and rapidly succumb to disease.106,107 Depletion of CD4+ T-cells causes reactivation of latent infection in mice,107 and increased pathology and bacterial burden during the first 8 weeks of infection in nonhuman primates (NHPs).108 The increased risk of TB disease due to decreased CD4+ T-cell number and function associated with HIV provides further evidence of a critical role for this cell type.109 CD8+ T-cells contribute to protective immunity through secretion of pro-inflammatory cytokines such as IFN-γ or by direct killing of M.tb-infected cells via granule-mediated functions.110 M.tb lipid antigens can also be presented to unconventional T-cells such as γδ T-cells, natural killer T cells, and mucosal-asso- ciated invariant T cells, stimulating effector functions that may be of importance.111
Upon recognition of M.tb-infected antigen presenting cells, CD4+ T-cells are primed as Th1 cells and become a primary source of IFN-γ, TNF-α, and interleukin (IL)-2. A central role for IFN-γ in activating macrophages to kill intracellular mycobacteria is well-established. Murine experiments demonstrated that IFN-γ KO mice are extremely susceptible to M.tb infection,112 and genetic studies of Mendelian susceptibility to mycobacterial disease have indicated defects in the IFN-γ/IL-12 signaling pathway.113 TNF-α, like IFN-γ, is involved in activation of bactericidal activity in infected macrophages. Treatment of mice with anti-TNF antibody results in fatal reactivation of persistent M.tb infection114; and the use of anti-TNF agents for inflammatory conditions such as rheumatoid arthritis has led to reactivation of LTBI.115 IL-2 directly promotes T-cell expansion and survival, whereas granulocyte-macrophage colony-stimulating factor does so via the modification of dendritic cell function; increasing IL-12 production, and expression of co-stimulatory molecules on the cell surface.116
Humoral immunity
The role of B-cells and antibodies in the immune response to M.tb has been elusive; recent evidence suggests they play a greater role than previously thought.117,118 Although M.tb studies in B-cell- deficient mice have had variable outcomes,119–121 B-cells are now thought to influence cytokine production, bacillary containment, and immunopathologic progression during M.tb infection. B-cells may modulate the T-cell response, participating in T-cell priming through antigen capture and presentation.122,123 Variable results from early serum therapy experiments led to a perceived minor role for antibodies. However, more recently monoclonal antibodies specific for mycobacterial components have been shown to protect mice against M.tb.124,125 Children with low levels of specific immunoglobulin G (IgG) were found to be at greater risk from disseminated TB, and low antibody titers to Ag85 complex antigens have been associated with a poor disease outcome.126,127 High titers of IgG against Ag85A were associated with reduced risk of developing TB disease in a case–control study in South African infants.128 Potential mechanisms of antibody-mediated immunity include modulation of the macrophage–pathogen interaction via FcR-mediated phagocytosis, augmentation of complementinduced killing, antibody dependent cell-mediated cytotoxicity, and mucosal protection.129
Correlates of protection studies have cast doubt on the sufficiency of the cell-mediated immune response to confer protection by vaccination.130 Although the vaccine candidate MVA85A was immunogenic, inducing a robust and durable polyfunctional CD4+ T-cell response, it did not demonstrate protective efficacy in a recent phase IIb trial.131 The key to a successful TB vaccine may lie in the harnessing of humoral immunity in concert with a more potent cell-mediated response.
TARGET POPULATIONS FOR
AN IMPROVED TB VACCINE
The populations most in need of a protective TB vaccine are those in which the burden of disease is highest and the public health impact of effective vaccination greatest. This includes infants, adolescents/young people, and HIV-infected adults. In high-bur- den countries, children contribute greater than 20% of the TB case load.132 They are at higher risk of developing TB disease after exposure compared with adults, with the greatest risk in those aged less than 2 years.133 In a study of age-related TB incidence in children in South Africa, TB incidence in children under 5 years of age was high (2.9%), and peaked at 12–23 months.28 Interestingly, TB disease is relatively uncommon in children aged 5–12 years, but the incidence rises again sharply in adolescence.134 Rates of smearpositive disease are highest in adolescents and young adults, and this is where the burden of transmission lies. This incidence peak also coincides with the most economically productive age, resulting in high-economic impact for endemic countries. Infection with HIV increases the risk of both new infections with M.tb and reactivation of latent M.tb infection by as much as 20% in highburden countries.109,135 Treatment with anti-retroviral therapy reduces this risk but not to the levels observed in HIV-negative individuals.136
In the adolescent/young adult and HIV-infected populations, a proportion of individuals will already be latently infected with M.tb. Thus a post-exposure vaccine designed to prevent reactivation of infection, and potentially eradicate it, would be highly desirable. It is unclear whether a vaccine designed to prevent primary disease would also be effective in this setting. Vaccines may also be designed as an immunotherapeutic to be administered as an adjunct to chemotherapy in patients with active disease, particularly in cases of drug-resistant TB. Several candidates in the current TB vaccine pipeline have been developed with this aim.137,138
NEW VACCINE APPROACHES
Given the protection conferred by BCG against severe and disseminated disease,34,35 there are significant ethical issues around withholding routine BCG immunization. This provides logistical challenges in the conduct of efficacy trials of new TB vaccines, particularly those designed to replace BCG. The two main approaches are to improve on BCG through genetic engineering of BCG or another mycobacteria (including M.tb itself), or a primeboost strategy in which a new vaccine is given at a later stage as a booster to BCG. Despite scientific progress and over a dozen

222 BCG and Other Vaccines
Table 12.2 Candidate TB vaccines currently in clinical development |
|
|
||
Strategy |
Vaccine |
Description |
Phase |
Ref. |
Replacing BCG |
|
|
|
|
Recombinant BCG |
VPM1002 |
BCG expressing listeriolysin and lacking urease C |
III |
142 |
strains |
|
|
|
|
Attenuated M.tb |
MTBVAC |
M.tb with deletion mutations in the virulence genes phoP |
IIa |
146–148 |
strains |
|
and fadD26 |
|
|
Other attenuated |
DAR-901 |
M. obuense |
IIb |
154 |
whole organism |
RUTI |
Fragmented M.tb; immunotherapeutic |
IIa |
137 |
mycobacteria |
MIP |
Heat-inactivated M. indicus pranii; immunotherapeutic |
III |
138,155,156 |
Boosting BCG with a subunit vaccine |
|
|
|
|
Protein/adjuvant |
M72/AS01 |
Fusion protein of Mtb39a and Mtb32a with AS01 adjuvant |
IIb |
157,160–164 |
candidates |
H4/IC31 (AERAS-404) |
Fusion protein of Ag85B and TB10.4 |
IIa |
57,168,169 |
|
H56/IC31 (AERAS-456) |
Fusion protein of Ag85B and ESAT-6 and the latency |
IIb |
170,171 |
|
|
antigen Rv2660 |
|
|
|
ID93+GLA-SE |
Fusion protein of Rv2608, Rv3619, Rv1813 and Rv3620 |
IIa |
172,173 |
|
|
formulated GLA-SE adjuvant |
|
|
Recombinant viral |
MVA85A |
MVA vector expressing AgA5A delivered by aerosol |
I |
185 |
vectors |
ChAdOx1.85A/ |
Chimpanzee adenovirus 5 expressing Ag85A prime, with |
I |
|
|
MVA85A |
an MVA85A boost |
|
|
|
Ad5Ag85A |
Human adenovirus 5 expressing Ag85A |
I |
174,186,187,246 |
|
TB/FLU-04L |
Live attenuated influenza A virus vector expressing |
IIa |
|
|
|
ESAT-6 and Ag85A |
|
|
vaccine candidates in the current clinical pipeline, few have progressed to efficacy trials and few new vaccines have been added in the last 5 years. The current lead candidates are reviewed later and summarized in Table 12.2.
Replacing BCG
Live attenuated whole-cell vaccines have the potential advantages over protein-adjuvant and viral-vectored subunit vaccines of a comprehensive antigen repertoire and greater similarity to natural infection. Such BCG replacements aim to improve on both efficacy (particularly in the developing world) and safety, permitting use in infants with HIV infection. Any BCG replacement must demonstrate non-inferiority to BCG in protection against other mycobacterial infections such as leprosy, and potential non-spe- cific effects in reducing all-cause mortality in infants, as well as efficacy against severe forms of TB and pulmonary disease.
RECOMBINANT BCG STRAINS
The two approaches for generating a genetically improved BCG strain are overexpression of immunodominant antigens and manipulation of antigen processing. The first strategy involves addition of antigens which BCG already expresses at a low level such as Ag85B in rBCG30,139 or addition of antigens that BCG does not currently express such as RD1 in BCG::RD1-2FG.140 rBCG VPM1002 employs the second strategy, expressing listeriolysin from Listeria monocytogenes and lacking urease C, with the aim of increasing acidification of the phagosome to enable enhanced membrane-perforating action of the listeriolysin. This is designed to increase endosomal escape of antigen, thus facilitating cross-priming and induction of a class I-restricted CD8+ T-cell response.141 VPM1002 has demonstrated clinical safety and
immunogenicity,142 and has entered into phase II clinical trials evaluating safety and immunogenicity in HIV-exposed infants, and as a method for preventing recurrence after initial successful TB treatment (NCT02391415 and NCT03152903).
AERAS-422 was designed to combine the two strategies, overexpressing Ag85A, Ag85B, and Rv3407, while also expressing perfringolysin (with the same aim as listeriolysin in VPM1002). Further development of this vaccine was terminated due to the reactivation of latent Varicella zoster infection in two subjects during phase I clinical trials.143 Of the three rBCG vaccines that have entered phase I trials (rBCG30, AERAS-422, and VPM1002), only VPM1002 remains in active development.
ATTENUATED M.TB STRAINS
An alternative approach is to use attenuated M.tb in which virulence-related genes have been deleted while conserving major immunodominant antigens such as early-secreted anti- gen-6 (ESAT-6) and culture filtrate protein-10 (CFP-10) that are absent in BCG. In light of safety concerns regarding advancing attenuated strains of M.tb to clinical evaluation (particularly in immunocompromised populations), two international meetings with regulators and vaccine developers coordinated by the WHO led to a set of recommendations on the construction and preclinical safety testing of these vaccines.144,145 MTBVAC, which contains two independent deletion mutations in the virulence genes phoP and fadD26, fulfills the Geneva consensus safety requirements and is the first of its kind to enter clinical trials. This vaccine demonstrated superior protection to BCG in guinea pigs and NHPs,146,147 and in a recent phase I clinical trial was found to be as safe as BCG, and comparably immunogenic.148 A phase Ib/IIa trial in adults with and without LTBI is underway (NCT02933281).
Книга в списке рекомендаций к покупке и прочтению сайта https://meduniver.com/

New vaccine approaches 223
OTHER ATTENUATED WHOLE ORGANISM MYCOBACTERIAL VACCINE CANDIDATES
SRL-172 is an inactivated whole cell non-tuberculous mycobacterial vaccine that has been evaluated in several clinical trials as an immunotherapeutic agent in combination with drug therapy.149 It was originally designated Mycobacterium vaccae by phenotypic methods, but has since been identified as Mycobacterium obuense. SRL-172 was shown to be safe and immunogenic in phase I and II studies in HIV-infected adults in Finland and Zambia.150,151 Although a phase III trial in South Africa found no efficacy in patients with newly diagnosed pulmonary TB,152 a trial in Tanzania indicated that boosting childhood BCG with five doses of M. vaccae provided 39% protection against definite TB in HIV-infected adults.153 However, there was no protection against clinical/probable TB and insufficient numbers of volunteers reached the primary endpoint of disseminated TB to power a comparison between the vaccine and placebo arms. DAR-901 is a new BCG booster vaccine manufactured from the same seed strain as SRL-172 using a new scalable method. In a phase I dose-escalation trial, DAR-901 was safe and well-tolerated at all dose levels and did not result in IGRA conversion.154 A phase IIb trial for the prevention of infection (POI) in adolescents is underway in Tanzania (NCT02712424).
RUTI is a polyantigenic liposomal vaccine made of detoxified fragmented M.tb cells that aims to improve outcomes in the treatment of both LTBI and TB disease and reduce antibiotic exposure. A phase IIa trial in South Africa demonstrated acceptable tolerability and good immunogenicity in HIV-infected and -uninfected subjects with LTBI following 1 month of isoniazid treatment.137 A phase IIa trial will assess safety and immunogenicity in patients with MDR-TB. Mycobacterium indicus pranii (MIP) (previously Mw) is another heat-inactivated mycobacterial strain being tested as a therapeutic agent. Although preclinical studies were promising,155 MIP had no immunotherapeutic effect on patients with tuberculosis pericarditis in a phase III clinical trial.138 However, the use of MIP in sepsis was associated with improved outcomes in a recent randomized trial156 and this vaccine has now progressed to a phase IIb trial in patients with severe sepsis (NCT02330432).
Boosting BCG with a subunit vaccine
An alternative approach to replacing BCG is to continue its administration at birth and develop a heterologous vaccine to be given at a later time with the aim of boosting the primed immune response. This would allow the benefits of BCG to be retained while increasing efficacy and durability. Such a vaccine could be given in infancy soon after BCG vaccination, in adolescence before the rise in TB incidence that occurs in young adults, or to HIV-infected adults at the time of diagnosis prior to the development of immunosuppression. A booster vaccine consists of one or more M.tb protein antigens (which must also be expressed in all strains of BCG) together with either an adjuvant or a recombinant viral vector delivery system.
PROTEIN/ADJUVANT VACCINE CANDIDATES
The most advanced protein/adjuvant candidate is M72/AS01; a fusion protein of the Mtb39a and Mtb32a antigens administered with the GSK proprietary AS01 adjuvant, which includes the
immunostimulants MPL and the saponin QS21 combined with liposomes.157 M72 administered with AS02 (a squalene-contain- ing emulsion formulation of the same immunostimulants as AS01) improved survival after M.tb challenge when co-adminis- tered with BCG in guinea pigs, enhanced immunogenicity but not efficacy in mice, and enhanced survival after challenge in BCGvaccinated NHPs.158,159 Clinical trials found that AS01 facilitated greater Th1 responses against both M.tb antigens than AS02 and this adjuvant was thus taken forward.157 M72/AS01 was well-toler- ated and induced robust polyfunctional M72-specific CD4+ T-cell and antibody responses in healthy adults, adolescents and BCGvaccinated infants.157,160–162 It was also safe and immunogenic in ART-stable and ART-naïve HIV-positive and HIV-negative adults in India.163 M72/AS01 has recently been evaluated in a phase IIb efficacy trial in Kenya, South Africa, and Zambia, and was found to provide 54% protection for latently infected adults against active TB disease.164
Three fusion protein/adjuvant vaccine candidates: Hybrid 1/ IC31, Hybrid 4/IC31, and Hybrid 56/IC31 were designed by the Statens Serum Institut (SSI) in Copenhagen. H1/IC31 contains the fusion protein of Ag85B and ESAT-6 adjuvanted to a system combining the antibacterial peptide KLK with a synthetic Tolllike receptor (TLR)-9 agonist (ODN1a). This vaccine was safe in healthy, prior, or latently TB-infected and HIV-infected adults, and induced a robust and durable CD4+ T-cell response.165–167 However, the inclusion of ESAT-6 potentially confounds the IGRA diagnostic assay which uses a T-cell response to ESAT-6 and CFP-10 as evidence of M.tb infection. Indeed, a recent study found that 17% of participants receiving high-dose H1/IC31 developed a positive response to the QuantiFERON TB Gold in-tube assay.165 H4/IC31 (AERAS-404) circumvents this issue by replacing ESAT-6 with TB10.4, while still demonstrating moderate protective efficacy in preclinical models.168,169 In a recent phase II trial conducted in South African adolescents, H4/IC31 showed no protection against initial infection, and a non-statistically significant trend toward reduced sustained M.tb infections.57
H56/IC31 is the newest candidate from this group, and contains the latency-associated antigen Rv2660 in addition to Ag85B and TB10.4. This vaccine is designed to also enhance protective immunity in individuals already latently infected with M.tb, to prevent reactivation and facilitate clearance of infection. H56/ IC31 was well-tolerated and immunogenic in NHP studies and showed protective efficacy against active TB disease and reactivation of latent infection.170 Phase I/IIa trials in healthy adults without or with LTBI demonstrated safety and immunogenicity. Interestingly, low-dose vaccination induced more polyfunctional and higher frequencies of specific CD4+ T-cells compared with high-dose vaccination.171 A phase II prevention-of-recurrence study is planned (NCT03512249).
ID93+GLA-SE consists of a fusion protein of four antigens (Rv2608, Rv3619, Rv1813, and Rv3620) formulated with the TLR adjuvant GLA-SE. This vaccine demonstrated protective immunity in mice and guinea pigs172 and was well-tolerated and immunogenic in a recent phase I dose-escalation study in healthy adults.173 Escalating doses induced similar antigen-specific CD4+ T-cell and humoral responses and showed an acceptable safety profile in BCG-vaccinated M.tb-infected individuals.173

224 BCG and Other Vaccines
RECOMBINANT VIRAL VECTORS
Live attenuated non-replicating viruses can be genetically engineered to deliver foreign antigens, and have the potential to engage the innate immune system and boost cellular immunity without the need for additional adjuvants. Most of the advanced vectored TB vaccine candidates are based on recombinant adenovirus or vaccinia virus. Other novel viral vectors include Sendai virus, lentivirus, parainfluenza virus 2, and influenza virus.
A recombinant modified vaccinia Ankara (MVA) vector expressing Ag85A was the first new subunit vaccine to enter clinical trials in 2002 and has been a leading candidate in the field for over a decade. MVA85A showed modest protection in preclinical animal models,147,175–177 and demonstrated safety and immunogenicity in phase I/IIa clinical trials.178–182 However, no improvement on protection provided by BCG alone was observed in a preventative pre-exposure phase IIb trial in South African infants.131 Preclinical studies have since demonstrated enhanced immunogenicity and/or protection when Ag85A is delivered intranasally or by aerosol.183,184 Clinical trials are now assessing the aerosol route of delivery of MVA85A with the aim of enhancing local protective immune responses at the primary site of infection. A phase I trial found that MVA85A delivered by aerosol was well-tolerated and elicited an immune response in the lungs that was superior to that induced by ID administration.185
Human adenovirus 5 (AdHu5) engineered to express Ag85A was found to be robustly protective against M.tb challenge in preclinical models, particularly when given via the intranasal route.186 It was also evaluated in a phase I clinical study and shown to be safe and immunogenic.187 However, one of the limitations of some virus-vectored vaccines is the presence of pre-existing antibodies induced by natural exposure which may compromise the potency of the vaccine and pose a safety concern when given to high-risk HIV-infected populations. In total, 45%–80% of adults have neutralizing antibody responses to AdHu5, depending on the region.188,189 One strategy to overcome anti-Ad immunity is the use of replication-defective chimpanzee-derived adenoviruses (AdCh), because neutralizing antibodies against these vectors are rarely found in humans190 and immunogenicity is comparable with that induced by human adenoviruses.191,192 In murine studies, AdCh68Ag85A induced superior T-cell responses and immune protection compared with its AdHu5 counterpart.193 An AdCh vector expressing Ag85A (ChAdOx1.85A) is currently being assessed in a prime-boost regimen with MVA85A in phase I trials (NCT01829490).
Antibodies to the less common AdHu35 virus are detected in just 5%–15% of adults.189 AERAS-402 is an AdHu35-vectored vaccine expressing a fusion protein of Ag85A, Ag85B, and TB10.4 which has demonstrated protective immune responses in mice.194 Safety and immunogenicity has also been assessed in clinical trials of healthy adults, adults with previous or active TBand HIV-infected adults.195–200 The vaccine was found to induce polyfunctional CD4+ T-cells and a potent CD8+ T-cell response, although recent analysis suggests that the polyfunctional T-cells do not necessarily recognize M.tb-infected targets.201 A phase IIb trial in BCG-vaccinated infants demonstrated an acceptable safety profile, but the trial was modified to remove the efficacy objective when the predefined immunogenicity target was not met.199
More recent studies have focussed on administering AERAS-402 by the aerosol route,202 and incorporating the vaccine in a primeboost strategy with MVA85A which has demonstrated increased frequency and durability of antigen-specific T-cell responses in phase I trials.203,204
TB/FLU-04L is a live-attenuated influenza A virus vector expressing the M.tb antigens ESAT-6 and Ag85A which is administered by the intranasal route. Preclinical studies have demonstrated safety, immunogenicity, and efficacy. In a phase I trial in BCG-vaccinated healthy volunteers, the vaccine was well-toler- ated and immunogenic with no infectious virus detected in nasal swabs by 5 days post-vaccination (NCT02501421).
CMV has recently been identified as a promising live attenuated viral vector due to its natural periodic reactivation, leading to intermittent re-stimulation of specific T-cells and maintenance of the effector cell population. The CMV/TB vaccine candidate expresses nine M.tb proteins representative of the acute-phase, latency, and resuscitation antigen classes. Preclinical studies in rhesus macaques demonstrated a 68% reduction in extent of M.tb infection and disease in the vaccinated animals compared with unvaccinated controls following M.tb challenge, with 14 out of 34 vaccinated animals showing no evidence of TB disease.205
CHALLENGES IN VACCINE
DEVELOPMENT
As the pathway for clinical development of new TB vaccines has become clearer, so have the challenges ahead. Major issues for the field over the next decade are the lack of validated immunological correlates of protection for down-selection of candidates going forward to efficacy testing, uncertainty regarding the predictive value of animal models, lack of a human challenge model, and lack of capacity to do and to fund phase IIb/III efficacy trials. Each of these issues is discussed briefly next.
Lack of a validated immunological correlate of protection
One of the greatest impediments to TB vaccine development is the lack of a validated immune correlate that reliably predicts vaccine efficacy. Such a biomarker would allow down-selection of candidates prior to entry into clinical trials and provide an alternative to clinical disease endpoints, shortening trials, and expediting vaccine development. The lack of an efficacious vaccine makes identification of a correlate of protection extremely challenging. However, biomarkers of reduced disease risk, for example in those who do not relapse after treatment or remain healthy with longstanding LTBI are important indicators of protective immunity and may aid in reducing the size and duration of clinical trials.
T-CELL AND B-CELL RESPONSES
IFN-γ has been widely used as the primary immunological readout in human trials, but studies in recent years have challenged the dogma of “the more IFN-γ the better.”206–209 In a 2-year fol- low-up study of BCG-immunized infants, the frequency and cytokine profile of mycobacteria-specific T-cells did not correlate
Книга в списке рекомендаций к покупке и прочтению сайта https://meduniver.com/
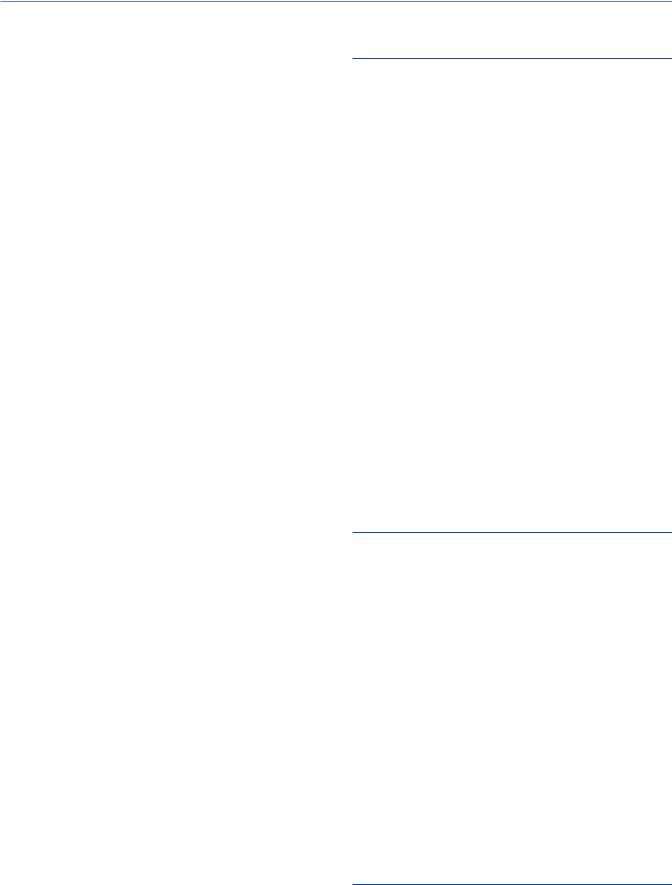
Challenges in vaccine development 225
with protection against TB.130 Despite promising results from other disease models and preclinical TB studies,210–213 polyfunctional T-cells also failed to associate with protective efficacy in this study.130 Interestingly, BCG-induced IFN-γ producing T-cells were associated with a reduced risk of TB disease in a recent case–control study in BCG-vaccinated South African infants, whereas activated CD4+ T-cells (expressing HLA-DR) were associated with increased risk.128 The causes of T-cell activation and impact on disease risk should be considered when designing and testing TB-vaccine candidates. B-cell correlates are understudied, but specific memory B-cells were found to be higher in BCGvaccinated compared with BCG-unvaccinated individuals,214 and higher concentrations of Ag85-specific IgG were associated with a reduced risk of TB in the infant case–control study.128
FUNCTIONAL MYCOBACTERIAL GROWTH INHIBITION ASSAYS
One alternative to hypothesis-driven measurement of predefined immune parameters is the use of unbiased in vitro mycobacterial growth inhibition assays (MGIAs). These are functional assays that take into account a range of immune mechanisms and their interactions.215 Early assays involved the use of whole blood inoculated with a luciferase-transfected BCG reporter strain,216 or co-cultures of stimulated or unstimulated lymphocytes added to infected monocytes.217–219 More recently, a simplified model based on direct infection of PBMC detected a BCG-induced improvement in control of mycobacterial growth in both UK adults and infants.220,221 Although growth inhibition was not associated with a reduced risk of TB disease in the South African infant case– control study,128 recent studies have demonstrated a correlation with protection from in vivo mycobacterial challenge in both humans and NHPs.247 Such assays also offer tractable systems for the investigation of underlying immune mechanisms, and to date control of mycobacterial growth in the direct PBMC MGIA has been associated with polyfunctional T-cells221 and trained innate immunity.222
GENE EXPRESSION SIGNATURES
Other unbiased approaches include global immune, gene expression, and proteomic profiling assays. Previous systems biology studies have reported signatures that discriminate TB disease from LTBI and other disease states.223–228 A study of latently infected South African adolescents with a 2-year follow-up identified a prospective 16-gene signature of risk of TB disease.229 This signature was validated in two independent cohorts of LTBI South African and Gambian adults, in which it predicted TB progression with a sensitivity of 53.7% (95% CI 42.6–64.3) and a specificity of 82.8% (95% CI 76.7–86).229 More recently, a four-gene signature derived from the samples in a South African and Gambian training set predicted progression up to 2 years before onset of disease in blinded test set samples, and was further validated in a cohort of adolescents with latent M.tb infection.230 A case–control study of more than 5,000 BCG vaccinated infants identified distinct host responses to vaccination observed, with two major clusters of gene expression demonstrating different myeloid and lymphoid activation and inflammatory patterns.231 This diversity should be taken into account in future vaccine development.
Predictive value of preclinical animal models
New candidate TB vaccines are currently evaluated using preclinical animal models; typically mice, guinea pigs, NHPs, and cattle. However, the relevance of these models in terms of predicting efficacy in humans is unclear. Vaccine efficacy in animal models, as determined by M.tb challenge studies, is defined as an improvement compared with control groups in a disease-related readout such as bacterial load, pathology score, or long-term survival. A vaccine may be considered to provide protection even if there are measurable bacteria or pathology in the organs or some animals do not survive. In humans, however, efficacy is defined as the prevention of TB disease using clinical endpoints.232 Furthermore, an artificial aerosol challenge is very different to natural transmission in humans, and the laboratory strains of M.tb commonly used are genetically dissimilar to clinical isolates,233 with much higher challenge doses employed. In addition to these fundamental differences in experimental design, animals are genetically distinct from humans, and the widely used mouse strains do not exhibit caseating granuloma formation: the hallmark of human disease.234 Cattle and NHPs are considered better models for human TB but their use is limited by cost and availability of animals and reagents.
There is yet to be an established link between a vaccine effect observed in animal models and human protection; recently, the modest protection conferred by MVA85A in preclinical studies did not translate into efficacy in humans.131,147,175,176 Only once we have an efficacious vaccine will it be possible to understand which animal models predict human efficacy, allowing those models to be optimized and best applied for subsequent development.
Lack of a human challenge model
Development and utilization of human challenge models has significantly accelerated vaccine development for a range of diseases including malaria, cholera, and typhoid.235–237 Such models offer the potential to down-select vaccine candidates at an early stage and to identify correlates of protective immunity. Although it is ethically unacceptable to infect volunteers with virulent M.tb, a live attenuated replicating mycobacterial strain such as BCG could offer a safe surrogate. Studies applying an intradermal BCG challenge model and quantifying mycobacteria from skin biopsies have demonstrated the ability to detect a degree of mycobacterial immunity in previously BCG-vaccinated individuals.238,239 A recent pilot study assessed a less invasive model measuring mycobacterial burden in swab specimens collected from the vaccination site.240 Further studies are underway, including the development of an aerosol BCG challenge model more representative of natural infection (NCT02709278). Fortune and colleagues are exploring strategies to generate a safe attenuated strain of M.tb that can be reproducibly cleared and not transmitted, and is detectable and quantifiable in vivo.241
Inadequate capacity to do and to fund phase IIb/III efficacy trials
The implementation of clinical trial sites for TB vaccine efficacy testing poses a major challenge to investigators. The requirements

226 BCG and Other Vaccines
include a robust clinical and laboratory infrastructure, established field resources, human skills and expertise, and known epidemiological data with which to power trials.242 There are currently very few sites worldwide that fulfill these criteria. The first site specifically developed for the evaluation of TB vaccines was the South African TB Vaccine Initiative (SATVI) site in Worcester, South Africa run jointly by the University of Cape Town. This was followed by a site at the Kenya Medical Research Institute/CDC field station. However, phase III trials will need to extend beyond the African subcontinent, and there is a major requirement for new sites in other TB-endemic regions such as Asia to allow multicenter licensure trials. Once sites have been established, expertise, capacity, and funding must be maintained between clinical trials to ensure that robust efficacy testing can commence without delay as soon as a candidate advances to this stage.
ALTERNATIVE ENDPOINTS
TB case accrual rates are the primary driver of the large size, duration, and cost of clinical efficacy trials. Trials designed with alternative, yet biologically meaningful endpoints that occur at higher rates than TB disease would allow smaller proof-of-concept trials to be conducted. Positive outcomes could then be used to justify subsequent acceleration into classical phase IIb and III prevention of disease trials. Such endpoints include POI and prevention of disease recurrence (POR). As described, recent studies suggest that
POI is feasible in principle,33 although one limitation is the lack of a gold standard for detecting M.tb infection. A recent trial of the AERAS-404 candidate employed an IGRA conversion POI primary endpoint, but did not demonstrate efficacy (NCT02075203). The high rate of TB disease recurrence and short time-frame (91% of relapses occur within the first year after completion of TB treatment243) would reduce follow-up time considerably in POR studies. Such studies would also establish proof of concept for vaccination as an adjunct to TB treatment, but perhaps set an unreasonably high bar for a prevention-of-disease vaccine and risk missing an impact on initial infection. The use of LTBI or other high risk volunteers would also accelerate clinical development; an approach currently being taken to evaluate the aforementioned M72/AS01 candidate (NCT01755598). The advantages and disadvantages of different clinical trial endpoints are summarized in Table 12.3.
CONCLUSIONS
After decades of neglect, TB vaccine development gained momentum in the 2000s but progress has slowed in recent years. It is hoped that the considerable advances made in discovery research and experimental medicine studies will feed into an improved pipeline in the future. However, the predictive value of animal models, correlates of protection, and human challenge models can only be
Table 12.3 Advantages and disadvantages of different clinical trial endpoints
Endpoint |
Advantages |
Prevention of disease (POD) |
Large public health impact by interrupting |
|
transmission |
|
Most widely accepted endpoint for TB vaccine |
|
registration |
|
Microbiologically confirmed TB disease endpoint |
|
accepted gold standard |
Disadvantages
Large sample sizes required
Long duration due to slow accrual of disease endpoints
Costly
Ability to prevent disease in uninfected and LTBI individuals may differ
Pre-existing anti-mycobacterial immunity may block vaccine effects in adult POD studies
Prevention of infection (POI) Infection rates higher than disease making trials smaller and shorter in duration
Potential marker of biological activity and gating strategy for advancement to POD trials
Ability to utilize existing infant vaccination programs for delivery
Lack of gold standard for detecting M.tb infection
POD trial still required
Phenomenon of reversion; need to capture sustained converters
Possibility that infection only prevented in those who would not otherwise progress to disease
POI may have smaller public health impact than POD Inadequate animal models to evaluate infection endpoint; limits advancement of POI candidates
Prevention of disease |
Disease endpoint accrual higher than in POD |
POD trial still required |
recurrence (POR) |
studies making trials smaller and shorter in |
|
|
duration |
|
|
Potential marker of biological activity and gating |
Risk of missing an impact on reducing initial infection |
|
strategy for advancement to POD trials |
|
|
May establish proof of concept for vaccination as |
|
|
adjunct to therapy |
|
Книга в списке рекомендаций к покупке и прочтению сайта https://meduniver.com/

References 227
validated by human efficacy data. The findings of the correlates of risk analysis using samples from the MVA85A phase IIb trial demonstrate how much can be learnt from such studies, regardless of efficacy outcome. It is essential to maintain momentum and funding if we are to attain the goals set out in the Global Plan to Stop TB and to significantly reduce the incidence of this devastating disease.
REFERENCES
1.Calmette A. Preventive vaccination against tuberculosis with BCG. Proc R Soc Med. 1931;24(11):1481–90.
2.Oettinger T, Jorgensen M, Ladefoged A, Haslov K, and Andersen
P.Development of the Mycobacterium bovis BCG vaccine: Review of the historical and biochemical evidence for a genealogical tree. Tuber Lung Dis. 1999;79(4):243–50.
3.Casanova J-L, Jouanguy E, Lamhamedi S, Blanche S, and Fischer
A.Immunological conditions of children with BCG disseminated infection. Lancet 1995;346(8974):581.
4.Hesseling AC et al. Bacille Calmette–Guérin vaccine-induced disease in HIV-infected and HIV-uninfected children. Clin Infect Dis. 2006;42(4):548–58.
5.Talbot EA, Perkins MD, Silva SF, and Frothingham R. Disseminated bacille Calmette–Guérin disease after vaccination: Case report and review. Clin Infect Dis. 1997;24(6):1139–46.
6.Lamm DL et al. Incidence and treatment of complications of bacillus Calmette–Guérin intravesical therapy in superficial bladder cancer. J Urol. 1992;147(3):596–600.
7.Brausi M et al. Side effects of Bacillus Calmette–Guérin (BCG) in the treatment of intermediateand high-risk Ta, T1 papillary carcinoma of the bladder: Results of the EORTC genito-urinary cancers group randomised phase 3 study comparing one-third dose with full dose and 1 year with 3 years of maintenance BCG. Eur Urol. 2014;65(1):69–76.
8.Lamm DL. Efficacy and safety of Bacille Calmette–Guérin immunotherapy in superficial bladder cancer. Clin Infect Dis. 2000;31(Suppl 3):S86–90.
9.Sylvester RJ, van der MA, and Lamm DL. Intravesical bacillus Calmette–Guérin reduces the risk of progression in patients with superficial bladder cancer: A meta-analysis of the published results of randomized clinical trials. J Urol. 2002;168(5):1964–70.
10.Nicol M, Eley B, Kibel M, and Hussey G. Intradermal BCG vac- cination—adverse reactions and their management. S Afr Med J. 2002;92(1):39–42.
11.Riordan A, Cole T, and Broomfield C. Fifteen-minute consultation: Bacillus Calmette–Guérin abscess and lymphadenitis. Arch Dis Child Educ Pract Ed. 2014;99(3):87–9.
12.Venkataraman A, Yusuff M, Liebeschuetz S, Riddell A, and Prendergast AJ. Management and outcome of Bacille Calmette– Guérin vaccine adverse reactions. Vaccine 2015;33(41):5470–4.
13.Gharehdaghi M, Hassani M, Ghodsi E, Khooei A, and Moayedpour
A.Bacille Calmette–Guérin Osteomyelitis. Arch Bone Jt Surg. 2015;3(4):291–5.
14.Macleod LC, Ngo TC, and Gonzalgo ML. Complications of intravesical Bacillus Calmette–Guérin. Can Urol Assoc J. 2014;8(7–8): E540–E4.
15.Eccles SR, and Mehta R. Disseminated BCG disease: A case report. Respir Med CME 2011;4(3):112–3.
16.Elkabani M, Greene JN, Vincent AL, Vanhook S, and Sandin RL. Disseminated Mycobacterium bovis after intravesicular Bacillus Calmette–Guérin treatments for bladder cancer. Cancer Control 2000;7(5):476–81.
17.Kesten S, Title L, Mullen B, and Grossman R. Pulmonary disease following intravesical BCG treatment. Thorax 1990;45(9):709–10.
18.Wayne LG. Microbiology of tubercle bacilli. Am Rev Respir Dis. 1982;125(3 Pt 2):31–41.
19.Ritz N, Tebruegge M, Connell TG, Sievers A, Robins-Browne R, and Curtis N. Susceptibility of Mycobacterium bovis BCG vaccine strains to antituberculous antibiotics. Antimicrob Agents Chemother. 2009;53(1):316–8.
20.Hesseling AC et al. Resistant Mycobacterium bovis Bacillus Calmette–Guérin disease: Implications for management of Bacillus Calmette–Guérin disease in human immunodeficiency virus-infected children. Pediatr Infect Dis J. 2004;23(5).
21.Sicevic S. Generalized BCG tuberculosis with fatal course in two sisters. Acta Paediatr Scand. 1972;61(2):178–84.
22.Boulware DR, Callens S, and Pahwa S. Pediatric HIV immune reconstitution inflammatory syndrome. Curr Opin HIV AIDS 2008;3(4):461–7.
23.Smith LL, Wright BL, and Buckley RH. Successful treatment of disseminated BCG in a patient with severe combined immunodeficiency. J Allergy Clin Immunol Pract. 2015;3(3):438–40.
24.Miret-Cuadras P, Pina-Gutierrez JM, and Juncosa S. Tuberculin reactivity in Bacillus Calmette–Guérin vaccinated subjects. Tuber Lung Dis. 1996;77(1):52–8.
25.Burl S et al. The tuberculin skin test (TST) is affected by recent BCG vaccination but not by exposure to non-tuberculosis mycobacteria (NTM) during early life. PLOS ONE 2010;5(8):e12287.
26.Sutherland I, and Lindgren I. The protective effect of BCG vaccination as indicated by autopsy studies. Tubercle 1979; 60(4):225–31.
27.Mahomed H et al. The impact of a change in bacille Calmette–
Guérin vaccine policy on tuberculosis incidence in children in Cape Town, South Africa. Pediatr Infect Dis J. 2006; 25(12):1167–72.
28.Moyo S et al. Age-related tuberculosis incidence and severity in children under 5 years of age in Cape Town, South Africa. Int J Tuberc Lung Dis. 2010;14(2):149–54.
29.Soysal A et al. Effect of BCG vaccination on risk of Mycobacterium tuberculosis infection in children with household tuberculosis contact: A prospective community-based study. Lancet 2005; 366(9495):1443–51.
30.Eisenhut M et al. BCG vaccination reduces risk of infection with Mycobacterium tuberculosis as detected by gamma interferon release assay. Vaccine 2009;27(44):6116–20.
31.Eriksen J et al. Protective effect of BCG vaccination in a nursery outbreak in 2009: Time to reconsider the vaccination threshold? Thorax 2010;65(12):1067.
32.Michelsen SW et al. The effectiveness of BCG vaccination in preventing Mycobacterium tuberculosis infection and disease in Greenland. Thorax 2014;69(9):851–6.
33.Roy A et al. Effect of BCG vaccination against Mycobacterium tuberculosis infection in children: Systematic review and metaanalysis. BMJ: Br Med J. 2014;349.
34.Trunz BB, Fine P, and Dye C. Effect of BCG vaccination on childhood tuberculous meningitis and miliary tuberculosis worldwide: A meta-analysis and assessment of cost-effectiveness. Lancet 2006;367(9517):1173–80.
35.Rodrigues LC, Diwan VK, and Wheeler JG. Protective effect of BCG against tuberculous meningitis and miliary tuberculosis: A meta-analysis. Int J Epidemiol. 1993;22(6):1154–8.
36.Colditz GA et al. Efficacy of BCG vaccine in the prevention of tuberculosis. Meta-analysis of the published literature. JAMA 1994;271(9):698–702.

228 BCG and Other Vaccines
37.Romanus V, Svensson A, and Hallander HO. The impact of changing BCG coverage on tuberculosis incidence in Swedish-born children between 1969 and 1989. Tuber Lung Dis. 1992;73(3):150–61.
38.Trnka L, Dankova D, and Svandova E. Six years’ experience with the discontinuation of BCG vaccination. 4. Protective effect of BCG vaccination against the Mycobacterium avium intracellulare complex. Tuber Lung Dis. 1994;75(5):348–52.
39.Fine PE. Variation in protection by BCG: Implications of and for heterologous immunity. Lancet 1995;346(8986):1339–45.
40.Tverdal A, and Funnemark E. Protective effect of BCG vaccination in Norway 1956–73. Tubercle 1988;69(2):119–23.
41.Hart PD, and Sutherland I. BCG and vole bacillus vaccines in the prevention of tuberculosis in adolescence and early adult life. Br Med J. 1977;2(6082):293–5.
42.Aronson JD. Protective vaccination against tuberculosis, with special reference to BCG vaccine. Minn Med. 1948;31(12):1336.
43.Hyge TV. The efficacy of BCG-vaccination; epidemic of tuberculosis in a State School, with an observation period of 12 years. Acta Tuberc Scand. 1956;32(2):89–107.
44.Baily GV. Tuberculosis prevention trial, Madras. Indian J Med Res. 1980;72(Suppl):1–74.
45.Ponnighaus JM et al. Efficacy of BCG vaccine against leprosy and tuberculosis in northern Malawi. Lancet 1992;339(8794):636–9.
46.Comstock GW, Woolpert SF, and Livesay VT. Tuberculosis studies in Muscogee County, Georgia. Twenty-year evaluation of a community trial of BCG vaccination. Public Health Rep. 1976;91(3):276–80.
47.Shapiro C et al. A case-control study of BCG and childhood tuberculosis in Cali, Colombia. Int J Epidemiol. 1985;14(3):441–6.
48.Sutherland I, and Springett VH. Effectiveness of BCG vaccination in England and Wales in 1983. Tubercle 1987;68(2):81–92.
49.Sterne JA, Rodrigues LC, and Guedes IN. Does the efficacy of BCG decline with time since vaccination? Int J Tuberc Lung Dis. 1998;2(3):200–7.
50.Nguipdop-Djomo P, Heldal E, Rodrigues LC, Abubakar I, and Mangtani P. Duration of BCG protection against tuberculosis and change in effectiveness with time since vaccination in Norway: A retrospective population-based cohort study. Lancet Infect Dis. 2016;16(2):219–26.
51.Barreto ML et al. Neonatal BCG protection against tuberculosis lasts for 20 years in Brazil. Int J Tuberc Lung Dis. 2005;9(10):1171–3.
52.Aronson NE et al. Long-term efficacy of BCG vaccine in American Indians and Alaska Natives: A 60-year follow-up study. JAMA 2004;291(17):2086–91.
53.Mangtani P et al. The duration of protection of school-aged BCG vaccination in England: A population-based case–control study. Int J Epidemiol. 2018;47(1):193–201.
54.Karonga Prevention Trial Group. Randomised controlled trial of single BCG, repeated BCG, or combined BCG and killed Mycobacterium leprae vaccine for prevention of leprosy and tuberculosis in Malawi. Lancet 1996;348(9019):17–24.
55.Rodrigues LC et al. Effect of BCG revaccination on incidence of tuberculosis in school-aged children in Brazil: The BCG-REVAC cluster-randomised trial. Lancet 2005;366(9493):1290–5.
56.Leung CC, Tam CM, Chan SL, Chan-Yeung M, Chan CK, and Chang KC. Efficacy of the BCG revaccination programme in a cohort given BCG vaccination at birth in Hong Kong. Int J Tuberc Lung Dis. 2001;5(8):717–23.
57.Nemes E et al. Prevention of M. tuberculosis infection with H4:IC31 vaccine or BCG revaccination. N Engl J Med. 2018;379(2):138–49.
58.Harboe M, Mshana RN, Closs O, Kronvall G, and Axelsen NH. Cross-reactions between mycobacteria. II. Crossed immunoelectrophoretic analysis of soluble antigens of BCG and comparison with other mycobacteria. Scand J Immunol. 1979;9(2):115–24.
59.Lombardi C, Pedrazzani ES, Pedrazzani JC, Filho PF, and Zicker
F.Protective efficacy of BCG against leprosy in Sao Paulo. Bull Pan Am Health Organ. 1996;30(1):24–30.
60.Merle CS, Cunha SS, and Rodrigues LC. BCG vaccination and leprosy protection: Review of current evidence and status of BCG in leprosy control. Expert Rev Vaccines 2010;9(2):209–22.
61.Setia MS, Steinmaus C, Ho CS, and Rutherford GW. The role of BCG in prevention of leprosy: A meta-analysis. Lancet Infect Dis. 2006;6(3):162–70.
62.Smith PG, Revill WD, Lukwago E, and Rykushin YP. The protective effect of BCG against Mycobacterium ulcerans disease: A controlled trial in an endemic area of Uganda. Trans R Soc Trop Med Hyg. 1976;70(5–6):449–57.
63.Noeske J et al. Buruli ulcer disease in Cameroon rediscovered. Am
JTrop Med Hyg. 2004;70(5):520–6.
64.Nackers F et al. BCG vaccine effectiveness against Buruli ulcer: A case-control study in Benin. Am J Trop Med Hyg. 2006;75(4):768–74.
65.Raghunathan PL et al. Risk factors for Buruli ulcer disease (Mycobacterium ulcerans infection): Results from a case-control study in Ghana. Clin Infect Dis. 2005;40(10):1445–53.
66.Orme IM, and Collins FM. Prophylactic effect in mice of BCG vaccination against non-tuberculous mycobacterial infections. Tubercle 1985;66(2):117–20.
67.Vaugelade J, Pinchinat S, Guiella G, Elguero E, and Simondon F. Non-specific effects of vaccination on child survival: Prospective cohort study in Burkina Faso. BMJ 2004;329(7478):1309.
68.Aaby P et al. Randomized trial of BCG vaccination at birth to low- birth-weight children: Beneficial nonspecific effects in the neonatal period? J Infect Dis. 2011;204(2):245–52.
69.de Castro MJ, Pardo-Seco J, and Martinon-Torres F. Nonspecific (heterologous) protection of neonatal BCG vaccination against hospitalization due to respiratory infection and sepsis. Clin Infect Dis. 2015;60(11):1611–9.
70.Garly ML et al. BCG scar and positive tuberculin reaction associated with reduced child mortality in West Africa. A non-specific beneficial effect of BCG? Vaccine 2003;21(21–22):2782–90.
71.Nankabirwa V, Tumwine JK, Mugaba PM, Tylleskär T, and Sommerfelt H. Child survival and BCG vaccination: A community based prospective cohort study in Uganda. BMC Public Health 2015;15(1):175.
72.Roth A et al. Tuberculin reaction, BCG scar, and lower female mortality. Epidemiology 2006;17(5):562–8.
73.Aaby P et al. Sex differential effects of routine immunizations and childhood survival in rural Malawi. Pediatr Infect Dis J. 2006;25(8):721–7.
74.Goodridge HS et al. Harnessing the beneficial heterologous effects of vaccination. Nat Rev Immunol. 2016;16(6):392–400.
75.Kleinnijenhuis J et al. Long-lasting effects of BCG vaccination on both heterologous Th1/Th17 responses and innate trained immunity. J Innate Immunity. 2014;6(2):152–8.
76.Bekkering S, Blok BA, Joosten LA, Riksen NP, van Crevel R, and Netea MG. In vitro experimental model of trained innate immunity in human primary monocytes. Clin Vaccine Immunol. 2016;23(12):926–33.
77.Haahr S et al. Non-specific effects of BCG vaccination on morbidity among children in Greenland: A population-based cohort study. Int J Epidemiol. 2016;45(6):2122–30.
78.Stensballe LG et al. BCG vaccination at birth and early childhood hospitalisation: A randomised clinical multicentre trial. Arch Dis Child. 2017;102(3):224.
79.Higgins JPT et al. Association of BCG, DTP, and measles containing vaccines with childhood mortality: Systematic review. BMJ 2016;355.
Книга в списке рекомендаций к покупке и прочтению сайта https://meduniver.com/

References 229
80.Raymond P. On the pathological relations between cancer and tuberculosis. Proc Soc Exp Biol Med. 1928;26(1):73–5.
81.Old LJ, Clarke DA, and Benacerraf B. Effect of Bacillus Calmette– Guérin infection on transplanted tumours in the mouse. Nature 1959;184(Suppl 5):291–2.
82.Carswell EA, Old LJ, Kassel RL, Green S, Fiore N, and Williamson B. An endotoxin-induced serum factor that causes necrosis of tumors. Proc Natl Acad Sci USA 1975;72(9):3666–70.
83.Hall MC et al. Guideline for the management of nonmuscle invasive bladder cancer (stages Ta, T1, and Tis): 2007 update. J Urol. 2007;178(6):2314–30.
84.Babjuk M et al. EAU guidelines on non-muscle-invasive urothelial carcinoma of the bladder, the 2011 update. Eur Urol. 2011;59(6):997–1008.
85.de Reijke TM et al. Bacillus Calmette–Guérin versus epirubicin for primary, secondary or concurrent carcinoma in situ of the bladder: Results of a European Organization for the Research and Treatment of Cancer-Genito-Urinary Group Phase III Trial (30906). J Urol. 2005;173(2):405–9.
86.Shelley MD, Court JB, Kynaston H, Wilt TJ, Coles B, and Mason
M. Intravesical bacillus Calmette–Guérin versus mitomycin C for Ta and T1 bladder cancer. Cochrane Database Syst Rev. 2003;(3):Cd003231.
87.Redelman-Sidi G, Glickman MS, and Bochner BH. The mechanism of action of BCG therapy for bladder cancer—a current perspective. Nat Rev Urol. 2014;11:153.
88.Kowalewicz-Kulbat M, and Locht C. BCG and protection against inflammatory and auto-immune diseases. Expert Rev Vaccines 2017;16(7):1–10.
89.Kühtreiber WM et al. Long-term reduction in hyperglycemia in advanced type 1 diabetes: The value of induced aerobic glycolysis with BCG vaccinations. NPJ Vaccines 2018;3(1):23.
90.Ritz N et al. The influence of bacille Calmette–Guérin vaccine strain on the immune response against tuberculosis: A randomized trial. Am J Respir Crit Care Med. 2012;185(2):213–22.
91.Kozak R, and Behr MA. Divergence of immunologic and protective responses of different BCG strains in a murine model. Vaccine 2011;29(7):1519–26.
92.Horwitz MA, Harth G, Dillon BJ, and Maslesa-Galic S. Commonly administered BCG strains including an evolutionarily early strain and evolutionarily late strains of disparate genealogy induce comparable protective immunity against tuberculosis. Vaccine 2009;27(3):441–5.
93.Ritz N, Hanekom WA, Robins-Browne R, Britton WJ, and Curtis N. Influence of BCG vaccine strain on the immune response and protection against tuberculosis. FEMS Microbiol Rev. 2008;32(5):821–41.
94.Edwards LB, Acquaviva FA, Livesay VT, Cross FW, and Palmer CE. An atlas of sensitivity to tuberculin, PPD-B, and histoplasmin in the United States. Am Rev Respir Dis. 1969;99(4 Suppl):1–132.
95.Black GF et al. BCG-induced increase in interferon-gamma response to mycobacterial antigens and efficacy of BCG vaccination in Malawi and the UK: Two randomised controlled studies. Lancet 2002;359(9315):1393–401.
96.Black GF et al. Patterns and implications of naturally acquired immune responses to environmental and tuberculous mycobacterial antigens in northern Malawi. J Infect Dis. 2001;184(3):322–9.
97.Palmer CE, and Long MW. Effects of infection with atypical mycobacteria on BCG vaccination and tuberculosis. Am Rev Respir Dis. 1966;94(4):553–68.
98.Brandt L et al. Failure of the Mycobacterium bovis BCG vaccine: Some species of environmental mycobacteria block multiplication of BCG and induction of protective immunity to tuberculosis. Infect Immun. 2002;70(2):672–8.
99.Floyd S et al. Kinetics of delayed-type hypersensitivity to tuberculin induced by Bacille Calmette–Guérin vaccination in northern Malawi. J Infect Dis. 2002;186(6):807–14.
100.Poyntz HC, Stylianou E, Griffiths KL, Marsay L, Checkley AM, and McShane H. Non-tuberculous mycobacteria have diverse effects on BCG efficacy against Mycobacterium tuberculosis. Tuberculosis (Edinburgh, Scotland) 2014;94(3):226–37.
101.Muller J et al. Cytomegalovirus infection is a risk factor for TB disease in infants. JCI Insight. 2019;4(23):e130090.
102.Ben-Smith A et al. Differences between naive and memory T cell phenotype in Malawian and UK adolescents: A role for cytomegalovirus? BMC Infect Dis. 2008;8:139.
103.Miles DJC, Gadama L, Gumbi A, Nyalo F, Makanani B, and Heyderman RS. Human immunodeficiency virus (HIV) infection during pregnancy induces CD4 T-cell differentiation and modulates responses to Bacille Calmette–Guérin (BCG) vaccine in HIVuninfected infants. Immunology 2010;129(3):446–54.
104.Elias D, Britton S, Aseffa A, Engers H, and Akuffo H. Poor immunogenicity of BCG in helminth infected population is associated with increased in vitro TGF-beta production. Vaccine 2008;26(31):3897–902.
105.Black S et al. Efficacy, safety and immunogenicity of heptavalent pneumococcal conjugate vaccine in children. Northern California Kaiser Permanente Vaccine Study Center Group. Pediatr Infect Dis J. 2000;19(3):187–95.
106.Caruso AM, Serbina N, Klein E, Triebold K, Bloom BR, and Flynn JL. Mice deficient in CD4 T cells have only transiently diminished levels of IFN-gamma, yet succumb to tuberculosis. J Immunol. 1999;162(9):5407–16.
107.Scanga CA et al. Depletion of CD4(+) T cells causes reactivation of murine persistent tuberculosis despite continued expression of interferon gamma and nitric oxide synthase 2. J Exp Med. 2000;192(3):347–58.
108.Lin PL et al. CD4 T cell depletion exacerbates acute Mycobacterium tuberculosis while reactivation of latent infection is dependent on severity of tissue depletion in cynomolgus macaques. AIDS Res Hum Retroviruses 2012;28(12):1693–702.
109.Geldmacher C, Zumla A, and Hoelscher M. Interaction between HIV and Mycobacterium tuberculosis: HIV-1-induced CD4 T-cell depletion and the development of active tuberculosis. Curr Opin HIV AIDS 2012;7(3):268–75.
110.Lin PL, and Flynn JL. CD8 T cells and Mycobacterium tuberculosis infection. Semin Immunopathol. 2015;37(3):239–49.
111.Huang S. Targeting innate-like T cells in tuberculosis. Front Immunol. 2016;7:594.
112.Flynn JL, Chan J, Triebold KJ, Dalton DK, Stewart TA, and Bloom BR. An essential role for interferon gamma in resistance to Mycobacterium tuberculosis infection. J Exp Med. 1993;178(6):2249–54.
113.Bustamante J, Boisson-Dupuis S, Abel L, and Casanova JL. Mendelian susceptibility to mycobacterial disease: Genetic, immunological, and clinical features of inborn errors of IFN-γ immunity. Semin Immunol. 2014;26(6):454–70.
114.Mohan VP et al. Effects of tumor necrosis factor alpha on host immune response in chronic persistent tuberculosis: Possible role for limiting pathology. Infect Immun. 2001;69(3):1847–55.
115.Lin PL, Plessner HL, Voitenok NN, and Flynn JL. Tumor necrosis factor and tuberculosis. J Investig Dermatol Symp Proc. 2007;12(1):22–5.
116.Nambiar JK, Ryan AA, Kong CU, Britton WJ, and Triccas JA. Modulation of pulmonary DC function by vaccine-encoded GM-CSF enhances protective immunity against Mycobacterium tuberculosis infection. Eur J Immunol. 2010;40(1):153–61.