
Mechanical Properties of Ceramics and Composites
.pdf10 |
Chapter 1 |
effects are important in the often substantial secondary effects on properties via associated microcracking.
Boundary, i.e. interfacial, pores and second phases between grains and particles or both are often present and can play significant roles in body properties via either or both of two avenues. The first is their often interactive effects impacting grain or particle sizes, shapes, and orientations and thus on properties dependent on these parameters. The second is the direct effect of such interfacial phases on properties by modifying the dependence of properties on unaltered grain or particle sizes, shapes, and orientations, e.g. in the extreme by dominating properties. Electrical breakdown across a range of temperatures and mechanical failure at higher temperatures are examples of the latter.
III.RANGE, CHARACTER, AND CHALLENGES OF GRAIN AND PARTICLE PARAMETERS IN MONOLITHIC AND COMPOSITE CERAMICS
A.General Issues and Challenges
Before proceeding to outline the methods of characterizing grain and particle structures, and associated measurements to understand the dependence properties of ceramic or composite ceramic bodies on them, it is important to recognize the challenges to such characterization and understanding. Basic challenges in normal characterization of grain and particle parameters are often significantly compounded primarily by two factors, which are also keys to the successful engineering of properties via fabrication-processing control of microstructure. The first is the variety of microstructures that can occur both locally and globally. The second is the particular sensitivity of some properties to local rather than general microstructure, especially larger grains, particles, or clusters of them along with their shape and orientation. However, the location of such microstructural heterogeneities in the body and their association with defects such as pores and cracks are also important. Properties particularly affected by such microstructural heterogeneities are those involving macrofracture, i.e. in approximate order of decreasing impact: tensile strength, electrical breakdown, fracture toughness, and compressive strength. Other properties sensitive to local microstructure, with or without local fracture, are hardness, wear, and erosion.
An important complication in determining grain or particle sizes occurs when the grains or particles are not nominally equiaxed. In such cases not only do both the shape and the orientation need to be characterized but also their often substantial impact on both the definition and the measurement of grain or particle size is a challenge. While such interactions pose a challenge for determining the average grain size, they can be of greater concern in describing the numerical and spatial distributions of grain sizes. In composites, another challenge is de-
Grain and Particle Effects on Ceramic Properties |
11 |
scribing the spatial distribution of the particles in the matrix. Interactions of size, shape, and orientation and the challenges of describing numerical, i.e. statistical, and spatial distributions of these have important parallels in describing some important variations of grain parameters.
Another issue is boundary, i.e. interfacial, phases, such as those that commonly result from impurities or residual densification additives. These often present measuring challenges, e.g. their presence and extent at low concentrations. At higher concentrations, both their uniformity and the contiguity of the grains or particles that such phases partially surround can be issues. The following section addresses grain and especially particle parameters in two-phase or multiphase ceramics, especially in designed composites, but natural composites such as whitewares and crystallized glasses are also addressed.
B.Grain Variations, Mainly Size in Nominally Single-Phase Ceramics
Significant challenges arise from grain variations within a given part as well as between different ones, which arise from both the fabrication method and its associated processing parameters, as well as from heterogeneities, e.g. impurities, in the body. A primary variation is commonly grain size, but interrelated factors of shape and orientation can also be important, with characterization to reflect more than one grain population, i.e. bior multimodal grain size distributions being a particular challenge. More extreme cases of bimodal grain distributions, which are those often of greater impact, are typically readily identifiable (e.g. Fig. 1.1). In such cases, the primary problem is commonly that of determining the average and variation (e.g. standard deviation) for each grain population. Accurately determining the volume fraction of each distribution and its spatial distribution are also challenging.
A common and important aspect of grain size variation in polycrystalline bodies is the heterogeneous distribution of larger grains having little or no relation to the normal grain size distribution(s). These larger grains, which are typically the result of local exaggerated grain growth, can be an isolated or dominant large grain (Fig. 1.2), or clusters of two to a few larger grains (Fig. 1.3), and occasionally clusters of several larger grains (Fig. 1.4) that can be of a wide variety of character. The grains in such clusters often have a common cause for their occurrence, in which case they have similar character. Of particular impact is the occurrence of much larger individual grains or of clusters of larger grains, which are of sufficient size to be all, or most, of fracture origins. Though this appears to require that they be associated with another defect such as a crack or pore to initiate fracture, this coincidence is common, but not universal. The impact of such isolated larger grains, or clusters of larger grains on fracture, scales inversely with the square root of their size, which can be an order of magnitude or more

12 |
Chapter 1 |
FIGURE 1.1 Clear, more extreme examples of bimodal grain structures seen on room-temperature fractures. (A) Pure, dense Al2O3 (TEM of fracture surface replica) showing two grain populations occurring as approximately interleaved ( vertical) laminations that are nominally perpendicular to the hot pressing direction. (B) Sintered ZrO2–8 wt% Y2O3 (SEM of fracture surface) showing a fine grain matrix with approximately random distribution of much larger grains singly or as clusters of two or three larger grains. Note in both cases that the smaller grains exhibit predominately intergranular and the larger ones predominately transgranular fracture.
larger than the average of the surrounding grains (e.g. Figures 1.2–1.4). The impact of such larger grains or grain clusters can be significant, especially on tensile strength, even if their occurrence is sparse, which makes determining their character and whether they were the actual source of failure challenging, especially if they are not specifically identified at fracture origins.
While the occurrence and character of larger isolated grains or grain clusters is quite variable, there are certain materials and processing factors that favor or exacerbate their occurrence and character. The material aspects entail the basic composition itself and local concentrations of additives or impurities, or sometimes deficiencies of them, especially additives. Consider first the basic material composition, where a common factor is crystal structure. Large grains or grain clusters are most common in some noncubic materials, e.g. CVD ZnSe [3, 4] (Fig. 1.3C), more extensively in hot pressed or sintered B4C [4–6] (Figures 1.2D, 1.3D) and Si3N4 [7–10] and especially in SiC [5, 6, 11], Al2O3 [3–5, 12–18], and beta aluminas [5, 19–21]. In Si3N4 they are commonly rod-shaped β grains often associated with local concentrations of oxide densification additives [8–10]. In SiC they are commonly long, narrow alpha platelets nucleated

Grain and Particle Effects on Ceramic Properties |
13 |
FIGURE 1.2 Examples of fracture origins from a single or dominate large grain seen on room temperature fractures. (A) and (B) Commercial sintered 96% alumina fracture origins from isolated larger grains at the flexure surface respectively at 22°C (376 MPa) and -196°C (593 MPa) after Gruver et al. [14]. (C) Origin from large grain at the flexure surface in pure hot pressed Al2O3 (396 MPa, giving KIC 2.7 MPa·m1/2). (D) Origin from a large grain internal from the flexure surface of a hot pressed B4C (<350 MPa at the grain). Smaller lighter area at the lower left side, and the larger one on the right of the large grain, are areas of some porosity and cracking. Note varying degrees of truncation of larger grains at the surface from machining.
in bodies of β grains during sintering, typically at temperatures of ≥ 2000°C, but they also occur as clusters in CVD SiC at much lower temperatures, as discussed below. Their lengths are often > 10 times their widths, with probable, but poorly characterized, thicknesses a fraction of their widths [11]. Larger isolated grains in beta alumina bodies are (usually thin) platelets, which are typical of such bodies and are attributed to exaggerated growth of grains of the same or modified beta aluminas [19–21], e.g. due to fluctuations in sodium content. Large grain character in Al2O3 is more varied, ranging from equiaxed to platelet or rod shapes. However, noticeable or significant exaggerated grain structures have apparently not been observed in some noncubic materials such as MgF2 and mullite, which commonly have finer grain structures. The frequency, extent, and character of larger isolated grains or heir clusters is generally less in cubic materials.
An important compositional impact on the occurrence and character of
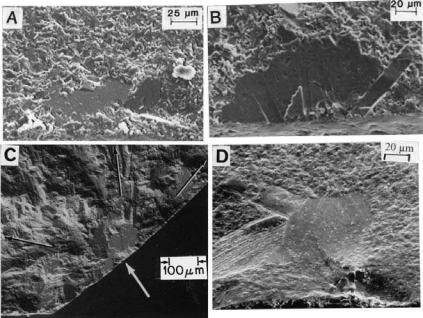
14 |
Chapter 1 |
FIGURE 1.3 Examples of a few larger grains at ceramic fracture origins in flexure at 22°C. (A) Pure hot pressed Al2O3 origin (483 MPa) via intergranular fracture from a large platelet grain in the plane of fracture, bottom center of photo, and two similar intermediate sized grains to the right, all three just inside the tensile surface and at progressively higher angles to the fracture surface. The third (right) grain is a white streak in this view, since it is seen nearly on edge, showing the thin platelet nature of many such grains in Al2O3. (B) Pure hot pressed Al2O3 origin from the tensile surface (405 MPa) from a large intergranularly fractured platelet grain in the plane of fracture, bottom center of micrograph, showing impressions of two or three intermediate sized tabular grains from the mating fracture surface and a transgranularly fractured grain (right). (C) CVD ZnSe flexure origin from a large grain in the plane of fracture at the specimen corner with an adjacent intermediate sized grain (both transgranularly fractured). (D) Hot pressed B4C origin (376 MPa) from a machining flaw (bottom center) and three to five larger grains above and to the left. Note light laminar striations on some of the grains indicating twinning and its interaction with the fracture.
larger grain structures in ceramics is the effects of additives, (local) impurities, or both. Again, a number of noncubic materials, especially Al2O3, show a greater extent and diversity of such effects, apparently reflecting significant anisotropy in crystal (grain) growth characteristics. Thus thin platelets are common in bodies of high purity Al2O3 hot pressed without MgO additions ([3–5, 12–16], for
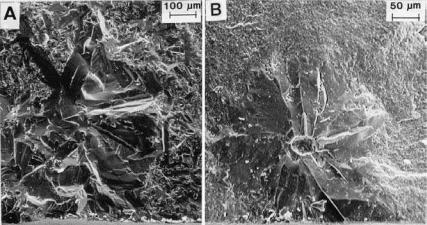
Grain and Particle Effects on Ceramic Properties |
15 |
FIGURE 1.4 Examples of flexure fracture origins from clusters of larger grains in alumina bodies in flexure at 22°C. (A) Pure hot pressed Al2O3 origin (443 MPa) from a cluster of tabular grains just inside the tensile surface, with mostly transgranular fracture and mixed radial and random orientations. (B) Experimental commercial sintered higher purity alumina origin (266 MPa) inside the tensile surface from a cluster of tabular grains radiating from a central pore.
grain growth control, which can eliminate them if it is homogeneously distributed; other grain variations can occur with heterogeneous MgO distribution [22]). Such platelet grains, which are apparently due to small amounts of Nabased impurities (whose volatilization may be inhibited in hot pressing) triggering formation and growth of beta alumina grains in the absence of MgO or other grain growth inhibition, are often primarily indicated by fracture occurring intergranularly between them and the adjoining finer grain structure (Fig. 1.3A). Thicker platelet or rod-shaped grains, as indicated by their transgranular fracture (Fig. 1.3B), and some nominally equiaxed (Fig. 1.2A) from similar or other sources, are also common. Many larger grains arise from impurities, especially when there is some radial growth pattern (Fig. 1.4), where the central void may be due to a volatilized impurity, e.g. sodium. In some cases larger grains associated with pores may not be immediately around the pore surface but somewhat into the surrounding solid, e.g. indicating diffusion of the species causing excess grain growth reaching the concentration range favoring such growth [22].
Much of the above occurrence of elongated grains or grain clusters reflects directional growth, e.g. from a local impurity source. However, more homogeneous impurity contents or excess additives commonly result in more equiaxed and homogeneous larger grain clusters This is commonly the case for the more limited occurrence of larger grains in cubic materials, e.g. MgO
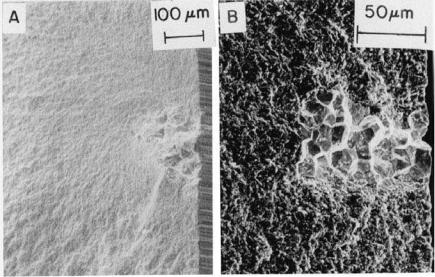
16 |
Chapter 1 |
FIGURE 1.5 Examples of clusters of larger equiaxed grains at flexure fracture origins in Si3N4 at 22°C. (A) Commercial hot pressed material (HS-110), origin (432 MPa) from a larger grain cluster associated with primarily excess Fe and secondarily Mn impurities. (B) Experimental hot pressed specimen origin (520 MPa) from a region of excess ZrO2 densification aid. (From Ref. 22. Published with the permission of Plenum Press.)
[4, 22]. However, similar effects also occur in noncubic materials, e.g. Si3N4 [22] (Fig. 1.5) and Al2O3(22).
The above common occurrence of variable and often significant roles that larger grains can play in mechanical failure raises important measurement issues. The arbitrary use of the size of the largest grains or particles for correlating with strength, as proposed by some, fails to recognize critical aspects of grains and particles as fracture origins, namely sufficient sizes and associated defects such as pores or cracks, which are discussed extensively in this book More fundamentally, effects of larger grains, as well as practical factors, argue against continuing the traditional dominance of obtaining grain and particle parameters from polished surfaces.
While microstructural measurements from polished surfaces can be of some use, their preparation and examination is far more time-consuming and costly than that of fracture surfaces, especially from property tests whose results are to be related to their microstructure. Fracture surfaces commonly reveal the microstructure, without etching, more clearly than polished surfaces, even with
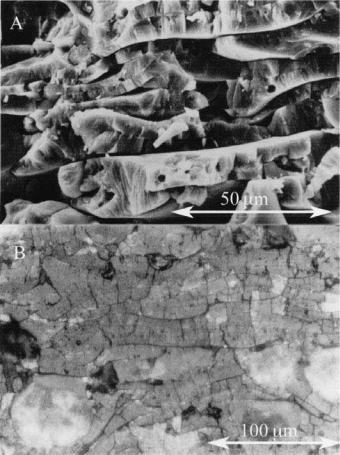
Grain and Particle Effects on Ceramic Properties |
17 |
good etching (e.g. Fig. 1.6). More fundamentally, polished surfaces fail to provide adequate information of two types. First, even with ideal polishing and etching there is essentially zero probability of such surfaces showing the extremes of grain structure that are often fracture origins. Such limitations are exacerbated when thin tabular or acicular grains are involved, since their
FIGURE 1.6 Comparison of essentially identical cross-sectional microstructures of the same Al2O3–wt% TiO2 plasma sprayed coating prepared by (A) fracture and
(B) conventional polishing and etching. Note much greater definition of the microstructure, especially the grain structure on the fracture surfaces, e.g. indicating a fine columnar grain structure within the individual splatted molten droplets. (From Ref. 23. Photos courtesy of Murakami et al.)
18 |
Chapter 1 |
observation and impact on grain size is dependent on their orientation relative to the surface of examination, with very low probability of a random polished surface giving results similar to that from fractures, especially fracture origins. The second and even greater deficiency of examining polished versus fracture surfaces for necessary grain structure information is that other key information is only available on the fracture surface. This includes whether the fracture origin was from an atypical area of grain structure of sufficient size and with necessary associated other defects (e.g. pores of cracks) to be the cause of failure, or whether failure was from the primary flaw population with little or no effect of grain structure variations. It also includes other important information such as the fracture mode, and especially for intergranular fracture, information on grain boundary character, e.g. phases and porosity.
C.Grain Variations, Mainly Shape and Orientation in Nominally Single-Phase Ceramics
The above outline of the occurrence of larger isolated grains or grain clusters has necessarily touched on grain shape and orientation, primarily in radial clusters. However, there are material and fabrication-processing factors that further affect either or both of these factors in a systematic fashion. Thus columnar, i.e. longer, typically rod-shaped, grains characteristically form normal to a solidification front with grain diameters and lengths typically inversely related to the speed of the solidification front and the density of nucleation sites for new grains. Such columnar grain structures occur in fusion cast refractories, especially near the mold surface where the solidification front is more uniform. The more rapid cooling, especially near the mold surface, commonly results in smaller grains there, though they are usually still substantially larger than grains in typical sintered bodies. More rapid cooling results in smaller size columnar grains, e.g. Fig. 1.7A. An extreme of the size/solidification speed is melt, e.g. plasma, sprayed ceramic coatings (Fig. 1.6B) [23]. Thus unless there are complications of other phases or formation of an intermediate amorphous phase, the molten droplets splatting onto the deposition surface directionally solidify normal to the surface, typically as fine columnar grains. A large-scale manifestation of such columnar structures and the common preferred crystal orientation in the columnar grains that typically accompanies it is the slow, controlled directional solidification of large polycrystalline ingots of cubic zirconia for the jewelry trade (Fig. 1.7B) [24]. Such grains can reach dimensions of 10 cm or more in large commercial ingots.
Vapor deposition processing, e.g. chemical vapor or physical vapor deposition (CVD or PVD), of coatings, or in the case of CVD also of bulk bodies, commonly results in various clustered grain and columnar structures that occur together or separately. The occurrence and character of these depends on deposi-

Grain and Particle Effects on Ceramic Properties |
19 |
FIGURE 1.7 Examples of columnar grains from directionally solidified melts of partially and fully stabilized zirconia bodies. (A) ZrO2 + 5 wt% Y2O3 sequentially solidified as thin layers on a solid surface (columnar grains form over the whole thickness of the layer with their length essentially being the layer thickness). (B) Examples of individual readily extractable grains (since the grains commonly are not bonded to one another, especially at such large grain sizes) from directionally solidified skull melts of differing w/o contents of Y2O3 (shown by each grain). (From Ref. 24)
tion rates (e.g. on pressure and temperature) and the phases involved [4, 25]. At high deposition rates, larger columnar grains result that are highly aligned axially (often with random radial crystal orientations), similar to those in directional solidification. (In such cases the ends of the columnar grains on the free surface of the deposit typically reflect single crystal faceting and are thus often referred to as faceted deposits or surfaces, e.g. in TiN [26].) Such columnar grains may have substantial aspect ratios, ranging from 1 to several-fold.
In the deposition range below that for producing larger aligned columnar grains, deposition often occurs by successively nucleating colonies of grains of similar orientation, especially axially with the growth direction. The colonies of grains of related orientation from a common nucleus, typically with higher axial than lateral growth rates, result in a knobby, bumpy, i.e. botryoidal or kidneylike free surface of the deposition (Fig. 1.8). Such colonies of grains with preferred axial orientations can occur with varying grain shapes, including blocky grains, but they commonly occur with bundles (colonies) of long, narrow columnar grains (Fig. 1.9). The colonies broaden out as growth occurs but then become constrained in their lateral growth by increasing competition with other colonies and in their axial growth by nucleation of new colonies, in all but very thin depositions.
Several factors should be noted about grain colonies. First, as a result of