
Mechanical Properties of Ceramics and Composites
.pdf120 |
Chapter 2 |
145.AV Virkar, GT Tennenhouse, RS Gordon. Hot-pressing of LiO2-stabilized β″-alu- mina. J Am Cer Soc 57(11):508, 1974.
146.AV Virkar, RS Gordon. Fracture properties of polycrystalline LiO2-stabilized β- alumina. J Am Cer Soc 60(1–2):58–61, 1977.
147.R Stevens. Strength and fracture mechanisms in beta-alumina. J Mat Sci 9:934–940, 1974.
148.YP Gupta, AT Santhanam. On cleavage surface energy of calcite crystals. Acta Metall. 17:419–424, 1969.
149.K Honma, M Yoshinaka, K Hirota, O Yamaguchi. Fabrication of calcite (CaC03) ceramics with high density. J Mat Sci Lett 17:745–746, 1998.
150.RA Schultz, MC Jensen, RC Bradt. Single crystal cleavage of brittle materials. Int J Fracture 65:291–312, 1994.
151.M Pardavi-Horváth. Microhardness and brittle fracture of garnet single crystals. J Mat Sci 19:1159–1170, 1984.
152.CP Chen, MH Leipold. Fracture toughness of silicon. Am Cer Soc Bull 59(4):469–472, 1980.
153.CP Chen, MH Leipold, Jr., D Helmreich. Fracture of directionally solidified multicrystalline silicon. J. Am Cer Soc 65(4):C-49, 1982.
154.M Iwasa, RC Bradt. Cleavage of natural and synthetic single crystal quartz. Mat Res Bull 22:1241–1248, 1987.
155.OO Adewoye. Anisotropic flow and fracture in beryl [Be3Al2(SiO3)6]. J Mat Sci 21:1161–1163, 1986.
156.M Sakai, RC Bradt, AS Kobayashi. The toughness of polycrystalline MgAl2O4. J Cer Soc Jpn, Intl Ed 96:510–515, 1988.
157.J Seidel, J Rodel. Measurement of crack tip toughness in alumina as a function of grain size. J Am Cer Soc 80(2):433–438, 1997.
158.WH Class, ES Machlin. Crack propagation method for measuring grain boundary energies in brittle materials. J Am Cer Soc 49(6):306–309, 1966.
159.J Tatami, T Harada, K Yasuda, Y Matsuo. Influence of twist angle on the toughness of (0001) twist boundary of alumina. Cer Eng Sci Proc 19:211–218, 1998.
160.HYB. Mar, WD Scott. Fracture induced in Al2O3 bicrystals by anisotropic thermal expansion. J Am Cer Soc 53(10):555–558, 1970.
161.WP Minnear, RC Bradt. Stoichiometry effects on the fracture of TiO2. J Am Cer Soc 63(9–10):485–490, 1980.
162.K Yasuda, S Ohsawa, Y Matsuo, S. Kimura. Influence of microcracking on frac-
ture toughness of TiO2 sintered body. J Jap Soc Mat Sci 41(463):482–488, 1992.
163.SW Freiman, L Chuck, JJ Mecholsky, DL Shellman, LJ Storz. Fracture Mechanisms in Lead Zirconate Titanate Ceramics. Fracture Mechanics of Ceramics 8, Microstructure, Methods, Design, and Fracture (R C Bradt, AG Evans, DPH Hasselman, FF Lange, eds.). Plenum Press, New York, 1986, pp. 175–185.
164.Y Ohya, Z-E Nakagawa, K Hamono. Crack healing and bending strength of aluminum titanate ceramics at high temperature. J Am Cer Soc 71(5):C-232–233, 1988.
165.EC Skaar, WC Croft. Thermal expansion of TiB2. J Am Cer Soc 56(1):45, 1973.
Grain Dependence of Microcracking, Crack Propagation |
121 |
166.AA Korneev, IT Ostapenko, MA Dolshek, AG Mironova, ND Rybal cenko, IA Lyashenko, VP Podtykan. Effect of annealing on the structure and properties of hot-pressed boron carbide. Poroshkovaya Metallurgiya 1 (349):9969–9972, 1972.
167.K Niihara, A Nakahira, T Hirai. The effect of stoichiometry on mechanical properties of boron carbide. J Am Cer Soc 74(1):C-13–14, 1984.
168.KA Schwetz, LS Sigl, L Pfau. Mechanical properties of injection molded B4C-C ceramics. J Solid State Chem. 133:68–76, 1997.
169.RW Rice, KR McKinney, CCm Wu, SW Freiman, WJ McDonough. Fracture energy of Si3N4. J. Mat Sci 20:1392–1406, 1985.
170.H-J Choi, YW Kim, J-G Lee. Effect of amount and composition of additives on the fracture toughness of silicon nitride. J Mat Sci Let 15:375–377, 1996.
171.JA Palm, CD Greskovitch. Thermomechanical properties of hot-pressed Si2.9Be0.1N3.8O0.2 ceramic. Am Cer Soc Bull 59(4):447–452, 1980.
172.M Shimada, M Koizumi, A Tanaka, T YYamada. Temperature dependence of KIC for high-pressure hot-pressed Si3N4 without additives. J Am Cer Soc 65(4):C-48, 1982.
173.I Tanaka, G Pezzotti, Y Miyamoto, T Okamoto. Fracture toughness of Si3N4 and its Si3N4 whisker composite without sintering aids. J Mat Sci 26:201–210, 1991.
174.K Niihara, T Hirai. Fractography of chemical vapor-deposited Si3N4. J Mat Sci, 13, 2385–2393 (1978).
175.SC Danforth, MH Richman. Strength and fracture toughness of reaction-bonded Si3N4. Am Cer Soc Bull 62(4):501–504, 1983.
176.E Tani, S Umebayashi, K Kishi, K Kobatashi, M Nishijima. Effect of size of grains
with fiber-Like structure of Si3N4 on fracture toughness. J Mat Sci Lett 4:1454–1456, 1985.
177.K Matsuhiro, T Takahashi. The effect of grain size on the toughness of sintered Si3N4. Cer Eng Sci Proc 10(7–8):807–816, 1989.
178.T Kawaehima, H Okamoto, H Yamamoto, A Kitamura. Grain size dependence of the fracture toughness of silicon nitride ceramics. J Cer Soc Jap, Intl Ed 99:310–313, 1991.
179.CMB Henderson, D Taylor. Thermal expansion of the nitrides and oxynitride of silicon in relation to their structures. Trans J Brit Cer Soc 74(2):49–53, 1975.
180.G Himsolt, H Knoch, H Huebner, FW Kleinlein. Mechanical properties of hotpressed silicon nitride with different grain structures. J Am Cer Soc 62(1–2):29–32, 1979.
181.AJ Pyzik, DF Carroll. Technology of self-reinforced silicon nitride. Ann Rev Mater Sci, Annual Reviews Inc. 24:189–214, 1994.
182.IM Peterson, T-Y Tien. Effect of the grain boundary thermal expansion coefficient on the fracture toughness in silicon nitride. J Am Cer Soc 78(9):2345–2352, 1995.
183.P Sajgalik, J Dusza, MJ Hoffmann. Relationship between microstructure, toughening mechanisms, and fracture toughness of reinforced silicon nitride ceramics. J Am Cer Soc 78(10):2619–2624, 1995.
184.T Ohji, K Hirao, S Kanzaki. Fracture resistance behavior of highly anisotropic silicon nitride. J Am Cer Soc 78(11):3125–3128, 1995.
185.J Wang, M Rainforth, R Stevens. The grain size dependence of the mechanical properties in TZP ceramics. Br Cer Trans 88(1):1–6, 1989.
122 |
Chapter 2 |
186.BA Cottom, MJ Mayo. Fracture toughness of nanocrystalline ZrO2-3 mol% Y2O3 determined by Vickers indentation. Scripta Mats 34 (5):809–814, 1996.
187.MV Swain. Grain-size dependence of toughness and transformability of 2 mol%- TZP ceramics. J Mat Sci Lett 5:1159–1162, 1986.
188.L Ruiz, MJ Readey. Effect of heat treatment on grain size, phase assemblage, and mechanical properties of 3 mol% Y-TZP. J Am Cer Soc 79(9):2331–2340, 1996.
189.GSAM Theunissen, JS Bouma, AJA Winnubst, AJ Burggraaf. Mechanical properties of ultra-fine grained zirconia ceramics. J Mat Sci 27:4429–4438, 1992.
190.P Duran, P Reico, JR Jurado, C Pascual, F Capel, C Moure. Y(E)-doped tetragonal zirconia polycrystalline solid electrolyte. J Mat Sci 24:708–716, 1989.
191.T Masaki. Mechanical properties of toughened ZrO2-Y2O3 ceramics. J Am Cer Soc 69(8):638–640, 1986.
192.J-G Duh, H-T Dai, B-S Chiou. Sintering, microstructure, hardness, and fracture toughness behavior of Y2O3-CeO2-ZrO2. J Am Cer Soc 71(10):813–819, 1988.
193.J Wang, XH Zheng, R Stevens. Fabrication and microstructure-mechanical property relationships in Ce-TZPs. J Mat Sci 27:5348–5356, 1992.
194.JG Duh, JU Wan. Developments in highly toughened CeO2-Y2O3-ZrO2 ceramic system. J Mat Sci 27:6197–6203, 1992.
195.PF Becher, KB Alexander, A Blier, SB Waters, WH Warwick. Influence of ZrO2 Grain size on the transformation response in the Al2O3-ZrO2 (12 mol% CeO2) system. J Am Cer Soc 76(3):657–663, 1995.
196.M Sakai, RC Bradt. The crack growth resistance curve of non-phase transforming ceramics. J Cer Soc Jpn, Inten Ed 96(8):801–809, 1988.
197.G Vekins, MF Ashby, PWR Beaumont. R-curve behavior of Al2O3 ceramics. Acta Met Mat 38(6):151–162, 1990.
198.BR Lawn, LM Braun, SJ Bennision, RF Cook. Reply to comment on role of grain size in the strength and R-curve properties of alumina. J Am Cer Soc 76(7):1900–1901, 1993.
199.R Steinbrech, R Khehans, W Schaarrwachter. Increase of crack resistance during slow crack growth in Al2O3 bend specimens. J Mat Sci 18:265–270, 1983.
200.C-W Li, DJ Lee, S-C Lui, J Goldacker. Relation Between strength, microstructure, and grain bridging in-situ reinforced silicon nitride. J Am Cer Soc 78(2):449–459, 1995.
201.A Okada, N Hiroshi. Subcritical crack growth in silicon nitride exhibiting R-curve. J Am Cer Soc 73(7):2095–2096, 1990.
202.N Hirosaki, Y Akimune, M Mitomo. Effect of grain growth of β-silicon nitride on strength, Weibull modules, and fracture toughness. J Am Cer Soc 76(7):1892–1894, 1993.
203.JA Salem, SR Choi, MR Freedman, MG Jenkins. Mechanical behavior and failure phenomenon of an in situ toughened silicon nitride. J Mat Sci 27:4421–4428, 1992.
204.M Sakai, J-I Yoshimura, Y Goto, M Ingaki. R-curve behavior of a polycrystalline graphite:microcracking and grain bridging in the wake region. J Am Cer Soc 71(8):609–616, 1988.
205.M Sakai, H Kurita. Size-effect on the fracture toughness and the R-curve of carbon materials. J Am Cer Soc 79(12):3177–3184, 1996.
Grain Dependence of Microcracking, Crack Propagation |
123 |
206.A Ghosh, KW White, MG Jenkins, AS Kobayashi, RC Bradt. Fracture resistance of a transparent magnesium aluminate spinel. J Am Cer Soc 74(7):1624–1630, 1991.
207.KW White, GP Kelkar. Evaluation of the crack bridging mechanism in a MgAl2O4spinel. J Am Cer Soc 74(70):1732–1734, 1991.
208.JC Hay, KW White. Grain-bridging mechanisms in monolithic alumina and spinel. J Am Cer Soc 76(7):1849–1854, 1993.
209.SK Lee, DK Kim, CH Kim. Flaw-tolerance and R-curve behavior of liquid-phase- sintered silicon carbides with different microstructures. J Am Cer Soc 78(1):65–70, 1995.
210.H Hubner, W Jillek. Sub-critical crack extension and crack resistance in polycrystalline alumina J Mat Sci 12:117–125, 1977.
211.L Ewart, S Suresh. Crack propagation in ceramics under cyclic loads. J Mat Sci 22:1173–1192, 1987.
212.G Choi, S Horibe. Static fatigue in ceramic materials:influence of an intergranular glassy phase and fracture toughness. J Mat Sci 28:5931–5936, 1993.
213.RW Steinbrech, R Khehans, W Schaarwachter. Increase of crack resistance during slow crack growth in Al2O3 bend specimens. J Mat Sci 18:265–270, 1983.
214.RW Steinbrech, O Schmenkel. Crack-resistance curves of surface cracks in alumina. J Am Cer Soc 71(5):C-271–273, 1988.
215.PF Becher, EY Sun, C-H Hsueh, KB Alexander, S-L Hwang, SB Waters, CG Westmoreland. Debonding of interfaces between beta-silicon nitride whiskers and Si- Al-Y oxynitride glasses. Acta Mater 44(10):3881–3893, 1996.
216.EY Sun, PF Becher, SB Waters, CH Hsueh, KP Plunckett, MJ Hoffmann. Control of Interface Fracture in Silicon Nitride Ceramics:Influence of Different Fare Earth Elements. In:Ceramic Microstructure:Control at the Atomic Level (AP Tomsia, A Glaeser, eds). Plenum Press, New York, 1998, pp. 779–786.
217.A Okada, N Hirosaki, M Yoshimura. Subcritical crack growth in sintered silicon nitride exhibiting a Rising R-Curve. J Am Cer Soc 73(7):2095–2096, 1990.
218.YW Kim, M Mitomo, N Hirosaki. R-curve behavior of sintered silicon nitride. J Mat Sci 30:4043–4048, 1995.
219.BN Cox. Extrinsic factors in the mechanics of bridged cracks. Acta Metall Mater 39(6):1189–1201, 1991.
220.J Rodel. Interaction between crack deflection and crack bridging. J Eur Cer Soc 10:143–150, 1992.
221.T Koyama, A Nishiyama, K Niihara. Effect of grain morphology and grain size on the mechanical properties of Al2O3 ceramics. J Mat Sci 29:3949–3954, 1994.
222.H-D Kim, I-S Lee, S-W Kang, J-W Ko. The formation of NaMg2Al15O25 in an α- Al2O3 matrix and its effect on the mechanical properties of alumina. J Mat Sci 29:4119–4124, 1994.
223.M Yasuoka, KME Brito, S Kanzaki. High-strength and high-fracture-toughness
ceramics in the Al2O3/LaAl11O18 systems. J Am Cer Soc 78(7):1853–1856, 1995.
224.BA Chandler, EC Duderstadt, JF White. Fabrication and properties of extruded and Sintered BeO. J Nuc Mat 8(3):329–347, 1963.
124 |
Chapter 2 |
225.RE Fryxell, BA Chandler. Creep, strength, expansion, and elastic moduli of sintered BeO as a function of grain size, porosity, and grain orientation. J Am Cer Soc 47(6):283–291, 1964.
226.JA Salem, JL Shannon, Jr., RC Bradt. Crack growth resistance in textured alumina. J Am Cer Soc 72(1):20–27, 1989.
227.M Iwasa, EC Liang, RC Bradt. Fracture of isotropic and textured Ba Hexaferrite. J Am Cer Soc 64(7):390–393, 1981.
228.G de With, N Hattu. Mechanical behavior of Sr-hexa ferrite. Proc Brit Cer Soc, No. 32, Engineering with Ceramics (RW Davidge, ed.), 191–198, 1982.
229.CCm Wu, KR McKinney, RW Rice. Strength and toughness of jade and related natural fibrous materials. J Mat Sci 25:2170–2174, 1990.
230.FF Lange. Relation between strength, fracture energy, and microstructure of hotpressed Si3N4. J Am Cer Soc 56(10):518–522, 1973.
231.FF Lange. Fracture toughness of Si3N4 as a function of the initial α-phase content. J Am Cer Soc 62(7–8):428–430, 1979.
232.JE Weston. Origin of strength anisotropi in hot-pressed silicon nitride. J Mat Sci 15:1568–1576, 1980.
233.M Ito, S Sakai, Y Yamauchi, T Ohoji, W. Kanematsu, S Ito. A study on strength
anisotropy of the fracture origin of HP-Si3N4. J Cer Soc Jpn, Int Ed 97:492–495, 1989.
234.F Lee, KJ Bowman. Texture and anisotropy in silicon Nitride. J Am Cer Soc 75(7):1748–1755, 1992.
235.Y Goto, A Tsuge, K Komeya. Preferred orientation and strength anisotropy of pressureless-sintered silicon nitride. J Eur Cer Soc 6:269–272, 1990.
236.W Kim, Y-W Kim, D Ho Cho. Texture and fracture toughness anisotropy in silicon carbide. J Am Cer Soc 81(6):1669–1672, 1998.
237.M Sakai, RC Bradt, DB Fischbach. Fracture toughness anisotropy of a pyrolytic carbon. J Mat Sci 21:1491–1501, 1986.
238.KD McHenery, BG Koepke. Electric field effects on subcritical crack growth in PZT. Fracture Mechanics of Ceramics 5 (RC Bradt, AG Evans, DPH Hasselman, FF Lange, eds.) Plenum Press, New York, 1983, pp. 337–352.
239.S-B Kim, D-Y Kim, J-J Kim, S-H Cho. Effect of grain size and poling on the fracture mode of lead zirconate titanate ceramics. J Am Cer Soc 73(1):561–563, 1990.
240.H-T Chung, B-C Shin, H-G Kim. Grain-size dependence of electrically induced microcracking in ferroelectric ceramics. J Am Cer Soc 72(2):327–329, 1989.
241.JW Halloran, EC Skaar. Poling stresses in lead zirconate titanate ceramics:part 1:measurement of residual poling stresses. Unpublished manuscript, 1981.
242.EC Skaar, JW Halloran. Poling stresses in lead zirconate titanate ceramics:part 2:the effect of poling stresses on strength. Unpublished manuscript, 1981.
243.Z Zhang, R Rai. The influence of grain size on ferroelastic toughening and piezoelectric behavior of PZT. J Am Cer Soc 78(12):3363–3368, 1995.
244.CS Lynch. Fracture of ferroelectric and relaxor electroceramics:influence of electric field. Acta Material. 46(2):599–608, 1998.
245.S Wan, KJ Bowman. Thermal depoling effects on anisotropy of lead zirconate titanate materials. J Am Cer Soc 81(10):2717–2720, 1998.
Grain Dependence of Microcracking, Crack Propagation |
125 |
246.QY Jiang, LE Cross. Effects of porosity on electric fatigue behavior in PLZT and PZT ferroelectric ceramics. J Mat Sci 28:4536–4543, 1993.
247.Q Jiang, W Cao, LE Cross. Electric fatigue in lead zirconate titanate ceramics. J Am Cer Soc 77(1):211–215, 1994.
248.GS White, AS Raynes, MD Vaudin, SW Freiman. Fracture behavior of cyclically loaded PZT. J Am Cer Soc 77(10):2603–2608, 1994.
249.MD Hill, GS White, CS Hwang. Cyclic damage in lead zirconate titanate. J Am Cer Soc 79(7):1915–1920, 1996.
250.ET Park, JL Routbort, Z Li, P Nash. Anisotropic microhardness in single-crystal and polycrystalline BaTiO3. J Mat Sci 33:669–673, 1998.
251.S Suresh. Fatigue crack growth in brittle materials. J Hard Mat 2(1–2):29–54, 1991.
252.RH Dauskardt. A frictional-wear mechanism for fatigue-crack growth in grain bridging ceramics. Acta Metall Mater 41(9):2756–2781, 1993.
253.MJ Hoffman, YW Mai, RH Dauskardt, J Ager, RO Ritchie. Grain size effects on cyclic fatigue and crack-growth resistance behavior of partially stabilized zirconia. J Mat Sci 30:3291–3299, 1995.
254.J Healy, AJ Bushby, YW Mai, AK Mukhopadhyav. Cyclic fatigue of long and short cracks in alumina. J Mat Sci 32:741–747, 1997.
255.H Kishimoto, A Ueno, S Okawara, H Kawamoto. Crack propagation behavior of polycrystalline alumina under static and cyclic load. J Am Cer Soc 77(5): 1324–1328, 1994.
256.DC Larson, LR Johnson, JW Adams, APS Teotia, LG Hill. Ceramic materials for advanced heat engines technical and economic evaluation. Noyes, Park Ridge, NJ, 1985.
257.RE Wright. Acoustic emission of aluminum titanate. J Am Cer Soc 55(1):54, 1972.
258.Y Ohya, Z-E Nakagawa, K Hamono. Grain-boundary microcracking due to thermal expansion anisotropy in aluminum titanate ceramics. J Am Cer Soc 70(8):C- 184–186, 1987.
259.LJ Graham, GA Alers. Microstructural aspects of acoustic emission generation in ceramics. Fracture Mechanics of Ceramics 1 (RC Bradt, DPH Hasselman, FF Lange, eds.). Plenum Press, New York, 1974, pp. 175–188.
260.Z Xiaoli, W Chongmin, Z Hongtu. Fracture toughness and acoustic emission in silicon nitride. J Mat Sci Lett 6:1459–1462, 1987.
261.DA Rega, DK Agrawal, C-Y Huang, HA McKinstry. Microstructure and microc-
racking behavior of barium zirconium phosphate (BaZr4P6O24) ceramics. J Mat Sci 27:2406–2412, 1992.
262.T Kishi, S Wakayama, S Kohara. Microfracture Process During Fracture Tough-
ness Testing in Al2O3 Ceramics Evaluated by AE Source Characterization. Fracture Mechanics of Ceramics (RC Bradt, AG Evans, DPH Hasselman, FF Lange, eds.). Plenum Press, New York, 1986, pp. 85–100.
263.C Sklarczyk. The acoustic emission analysis of the crack process in alumina. J Eur Cer Soc 9:427–435, 1992.
264.LC Sawyer, M Jamieson, D Brikowski, MI Haider, RT Chen. Strength, structure, and fracture properties of ceramic fibers produced from polymeric precursors. J Am Cer Soc 79(11):798–810, 1987.
126 |
Chapter 2 |
265.RW Rice. Fractographic determination of KIC and effects of microstructural stresses in ceramics. fractography of glasses and ceramics, ceramic trans. 17 (JR Varner, VD Frechette eds.). Am. Cer. Soc., Westerville, OH, 1991, pp. 509–545.
266.Vega-Boddio, J Schweitz, O Vingsbo. Surface energy measurements from Griffith cracks in boron Fibers. J Mat Sci 12:1692–1693, 1977.
267.J Lipowitz, JA Rabe, RM Salinger. Ceramic fibers derived from organosilicon polymers. Handbook of Fiber Science and Technology:Vol. III High Technology Fibers, Part C, Chapter 4 (M Lewin, J Preston, eds.). Marcel Dekker, New York, 1993, pp. 207–273.
268.MAH Donners. Fracture of MnZn ferrites. Proefschrift, Technische Universiteit Eindhoven, 1999.
269.K Tanaka, Y Kitahara, Y Ichinose, T Iimura. Fracture analysis of single crystal manganese zinc ferrites using indentation flaws. Acta Metal 32(1):1719–1729, 1984.
270.JJ Swab, JC LaSalvia, GA Gilde, PJ Patel, MJ Motyka. Transparent armor ceramics:AION and spinel. Cer Eng Sci Proc to be pub. in 2000.
271.S Park, C-T Sun. Fracture criteria for fiezoelectric ceramics. J Am Cer Soc 78(6): 1475–1480, 1995.
272.R Fu, T-Y Zhang. Effects of an electric field on the fracture toughness of poled lead zirconate titanate ceramics. To be published in J Am Cer Soc
273.B Rockower, E Hall. Investigation of Physical Properties of Beryllium Oxide as a Material for Integrating Gyros, MIT Instrumentation Lab. Report R-560, 9/1966, presented at the 1966 Fall Meeting. Electronics Div Am Cer Soc.
274.A G Imgram, M E Hoskins, J H Sovick, R E Maringer, F C Holden. Study of Microplastic Properties and Dimensional Stability of Materials. Battelle Mem. Inst. Tech. Report AFML-TR-67-232, Part II, for Air Force contract AF 33(615)-5218, Project 7351, 8/1968.
275.J W Moberly. Tensile Microstrain Properties of Telescope Mirror Materials. Stanford Res. Inst. Final Report for NASA contract NAS1-9982, 6/1971.

3
Grain Dependence of Ceramic
Tensile Strengths at 22°C
I.INTRODUCTION
A.Background
It has been known for years that grain effects play an important role in the tensile, hence flexure, strength (σ) of ceramics. Since grain size (G) is the most important and pervasive variable in both improving and understanding tensile strength, various G functions have been used to show strength decreases with increasing G. Some investigators opted for log σ vs. log G plots, e.g. Knudsen [1] but this compresses data at large G (obscuring important σ–G changes there). An inverse G function is preferred, since this allows use of data for all grain sizes, including single crystals (G = ∞), though with some large-G data compression. For mechanistic reasons discussed below, plotting σ vs. G-1/2 has become standard, but there is still some confusion and uncertainty concerning specific parameters and interpretations. Related issues of using average or maximum G values and branch intersections and slopes, particularly at finer G, whose clarification [2, 3] is addressed later, need broader recognition and use. The volume fraction porosity (P) and the shape and spatial variations of porosity, grains, and other phases can be important, particularly in view of the challenge of obtaining a significant range of specimens in which G is the primary factor determining σ.
Before proceeding to discussing mechanisms and presentation and evaluation of data it is useful to consider briefly the history of investigating and understanding grain size effects on the strength behavior of ceramics. To obtain reasonable data several limitations had to be overcome, often in an iterative fash-
127
128 |
Chapter 3 |
ion, namely effects of second, e.g. glassy, phases in many oxide ceramics such as Al2O3, and varying pore and flaw populations, and their interactions, and limitations and variations of grain size, shape, and orientation. Problems of these limitations were compounded by many studies focusing on one particular mechanism or one particular aspect of a variable, e.g. G distribution, often with a limited range of testing and evaluation, and failing to apply some necessary basic tools, especially fractography. An important step was sorting out the main porosity effects [1–6], thus enhancing identification of grain size effects.
Another major help was improvements in raw materials and processing, especially the introduction and increasing use of hot pressing, to give bodies with little or no porosity and second phases with either finer or larger G [7–11], thus better defining both branches (Fig. 3.1). Larger G behavior generally approximated a G -1/2 dependence of strength, suggesting grains being the flaws (recognizing that flaw size, c, is measured by a radius and G by a diameter). However, this relation must be limited, since it implies strength extrapolating to 0 at G = ∞, neglecting single crystal strengths generally being similar to many, and higher than some, polycrystalline bodies, as is discussed later. Finer grain samples typically showed some, but much less, grain size dependence than larger grain samples, i.e. laying mostly or completely along the finer grain branch (Fig. 3.1), hence projecting to a positive strength intercept at G = ∞ (i.e. G-1/2 = 0), commonly of the order of magnitude as for single crystal specimens with the same surface finish. The combination of such a positive intercept along with increasing observations of slip or twinning on a micro-, or in some cases a macro-, scale suggested possible microplastic crack nucleation, growth, or both. Carniglia’s surveys [8, 9] showed that when a sufficient G range was covered, both regimes of behavior were typically observed as a continuous function of G, showing finer G strengths decreasing with increasing G, extrapolated to σ > 0 at G = ∞, i.e. at G-1/2 = 0. Intermediate to large G samples showed greater σ decrease with increasing G, generally indicating extrapolation to σ 0 at G -1/2 = 0.
Carniglia suggested that the failure mechanism for the finer grain branch was microplasticity, hence referring to this as the Petch or Hall–Petch regime, and that for the large G branch it was Griffith flaw failure with the flaw dimensions being those of the grain size. The change between mechanisms was attributed to stresses for failure from flaws being higher than the stresses to activate microplastic failure at fine G, until G became sufficiently large to allow flaw failure at stresses below those needed for microplasticity. This provided not only a possible explanation for the two-branch character of the σ – G-1/2 plots commonly observed but also for variability in the intersection of the σ – G-1/2 branches. Thus, as the quality of surface finish improved in specimens lacking other major sources of flaws e.g., pores, the larger G branch would extend to finer G before microplasticity took over. A few investigators, such as Kirchner and Gruver [10] and Rice [3, 11–13], suggested contributions of other intrinsic
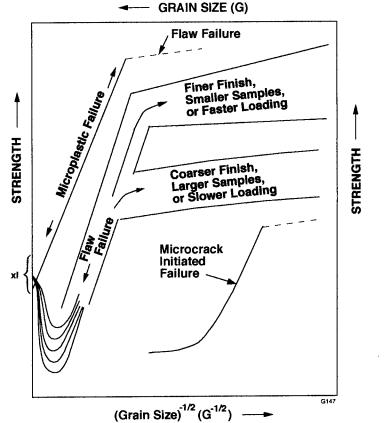
Grain Dependence of Ceramic Tensile Strengths at ~ 22°C |
129 |
FIGURE 3.1 Schematic of the preexisting flaw model showing the larger G branch with strengths decreasing rapidly with increased G, falling below single crystal strengths followed by (test and specimen dependent) variable reversal toward single crystal strengths. Finer G branches have lower slopes, giving less strength increases with decreasing G, and meet the larger G branch when c G/2. Since the finer G branch has flaws > G, i.e. not constrained by G, it can be more variable, e.g. wider, or may consist of different flaw populations, hence separate branches. Microcrack and microplastic variations shown illustrate similar rates of σ changes, e.g. an initially rapid decrease with the onset of substantial microcracking and then saturation. However, both models are shown at arbitrary strength levels relative to the preexisting flaw model.