
Drug Targeting Organ-Specific Strategies
.pdf74 3 Pulmonary Drug Delivery: Delivery To and Through the Lung
the correct size distribution during adequate inhalation. The inspiratory flow as the driving force for discharge of the dose system, fine particle generation during powder disintegration and particle deposition in the respiratory tract is one of the most important parameters. If the patient is unable to achieve the threshold values (for the relevant flow parameters) for good inhaler performance, the fine particle fraction will be too low for adequate efficacy. If the flow rate is too high, a substantial loss of the fine particle dose by inertial deposition in the oropharynx must be expected.
The nature of the flow curve achieved, depends on three different factors: the instructions given to the patient, the patient’s effort following these instruction and the resistance to airflow of the DPI (Figure 3.4). Most instructions for use of DPIs prescribe forceful and deep inhalation. Patient interpretation of these instructions often vary considerably. Patient variables also include age, gender, condition and clinical assessment. In Section 3.9 the attainable pressure drops across external resistances as a function of clinical condition will be discussed more in detail. The DPI’s resistance is a consequence of its design. Narrow channels in the dose system and the disintegration principle in addition to turbulent air zones, increase the resistance and reduce the attainable peak flow rate through the device. This is an advantage from the deposition point of view. High resistance DPIs generally have a high disintegration efficacy and do not require high flow rates to achieve an acceptable dose of fine particles. Recently, a multi-dose dry powder inhaler has been introduced (Sofotec Novolizer™) in which the resistance can be controlled over a certain range by means of a sheath flow around the aerosol cloud, without changing the fine particle output [55].
3.8 Airflow Resistance
The underpressure created in the respiratory tract is the driving force for the airflow through an inhalation device. The attainable underpressure and the rate of the airflow both depend on the total resistance in the airways and inhaler. The pressure drop achieved during inhalation is furthermore a function of the anatomy of the lungs, the effort made by the patient, pathological factors and the presence of exacerbations (e.g. in case of asthma).
A large proportion of the airflow resistance in the airways (internal resistance: Ri) is offered by the upper respiratory tract in which the airflow is already turbulent at relatively low flow rates of 30 to 40 l min–1 (RE > 2000: see also Section 3.2.1). During quiet mouth breathing, the mouth, pharynx, larynx and trachea account for 20–30% of total airway resistance. The same region contributes as much as 50% to total resistance during heavy breathing however. In the small peripheral airways (those less than 2 mm in diameter), resistance is quite low and the contribution to Ri is not more than 10–20% [21].
For nebulizers and MDIs, the external resistance (RE) is quite low. Different approaches have been made to describe the external airflow resistance of DPIs. Olsson and Asking [99] derived an empirical relationship between flow rate (Φ) and pressure drop (∆P), ∆P = C.Φ1.9, for a number of inhalers (such as Rotahaler™, Spinhaler™ and Turbuhaler™) in which they define the proportionality coefficient (C) as the airflow resistance. This relationship differs only slightly from the general (theoretical) equation for orifice types of flow constrictions:
ΦV = Fu(A) x (2∆P/ρA)0.5 |
(3.2) |
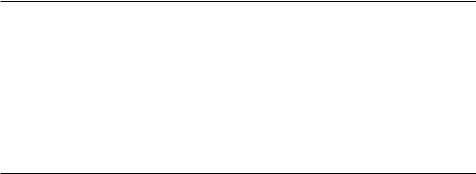
3.9 Inspiratory Pressure and Relevant Flow Parameters |
75 |
where ΦV is the volumetric flow rate, ρA is the density of the air (upstream of the flow constriction) and Fu(A) is a function of the cross-section of the flow constriction. Several authors use a simplification of this formula, written in terms of driving force (√∆P), airflow resistance (RE) and volumetric airflow (ΦV) (e.g. references [22,100]).
√∆P = RE x ΦV |
(3.2a) |
In this equation, RE is the reciprocal of Fu(A) x √(2/ρA). If there is no interaction between the flow through the inhaler and the flow in the respiratory tract, total resistance (RTOT) may be written as RTOT2 = RI2 + RE2. It has been reported that a high external resistance influences the shape and width of the human pharynx and larynx in a manner favourable for deep lung deposition [101].
The resistance of dry powder inhalers can be calculated by measuring the volumetric flow rate and the pressure drop across the device simultaneously and applying Eq. 3.2a for the calculation. Values for some marketed DPIs are given in Table 3.7, showing that there are remarkable differences between devices.
Table 3.7. Airflow resistances of some marketed dry powder inhalers in kPa0.5 min l–1.
Reference |
[100] |
[144] |
[145] |
Mean |
|
|
|
|
|
Glaxo Rotahaler |
0.013 |
0.013 |
0.014 |
0.0133 |
Fisons Spinhaler |
0.016 |
0.015 |
0.015 |
0.0153 |
Pharbita Cyclohalera |
0.017 |
0.018 |
0.018 |
0.0177 |
Sofotec Novolizer |
– |
– |
0.026 |
0.0260 |
Glaxo Diskhalerc |
0.021 |
0.018 |
0.030 |
0.0230 |
Glaxo Diskus |
– |
– |
0.032 |
0.0320 |
Astra Turbuhaler |
0.031 |
0.039 |
0.040 |
0.0367 |
Inhalator Ingelheimb |
0.056 |
0.053 |
0.048–0.058 |
0.0540 |
Chiesi Inhaler |
– |
0.093 |
– |
0.0930 |
aAlso known as ISF inhaler and Novartis (Foradil) Inhaler.
bThe range in reference [145] is a consequence of poor reproducibility in capsule piercing.
cThe spread for the Glaxo Diskhaler may come from the use of two different devices: four-dose [145] and eight-dose [100, 144].
1 technical bar equals 1000 mbar = 105 Pa (N m-2 or kg s-2 m-1) = 102 kPa
Resistances have been (re-)calculated for standard liters per minute (at 20 °C: LS min-1 = 1,0733*LN min–1).
3.9 Inspiratory Pressure and Relevant Flow Parameters
Several authors measured attainable pressure drops as a function of the external resistance for different groups of volunteers. Healthy male subjects (during maximal inspiration) are able to create a pressure drop (on average) of 6.7 kPa through an airflow resistance of 0.038 kPa0.5 min l–1, (which is in the range of that of marketed DPIs, see Table 3.7), whereas females are able to create a pressure drop of 3.8 kPa under the same resistance [22]. Differences between different groups of patients depend on the degree to which pulmonary func-
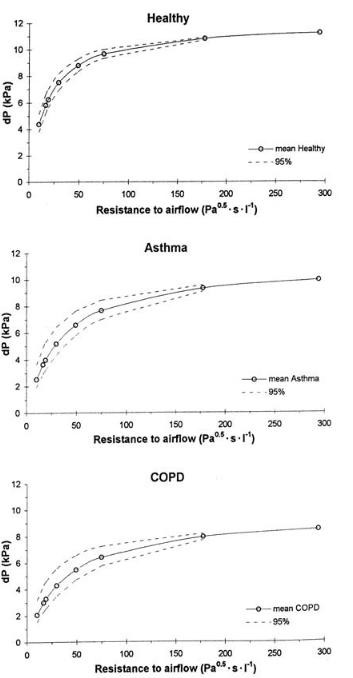
76 3 Pulmonary Drug Delivery: Delivery To and Through the Lung
Figure 3.5. The effect of external resistance and clinical conditions on the pressure drop generated at maximum inhalation effort. The area between the dashed lines represents the 95% confidence interval.
3.9 Inspiratory Pressure and Relevant Flow Parameters |
77 |
tion has been deteriorated. However, pulmonary obstructions usually restrict the expiratory performance rather than the inhalation manoeuvre (e.g. [102,103]). Therefore, maximal inspiratory pressure (MIP) values of asthmatic and COPD patients can be of the same order of magnitude as that of healthy subjects [104]. Only severe COPD patients may not be able to generate MIPs higher than 1.5–2.0 kPa. The effect of airflow resistance and clinical condition on the generated pressure drop across an external airflow resistance is summarized in Figure 3.5 (data derived from reference [104]). The data show that in vitro testing of dry powder inhalers at only 4 kPa, as prescribed by various guidelines, is inadequate for the prediction of their performance in practice.
Attained flow parameters during inhalation can either be calculated (Eq. 3.1) from recorded pressure drop curves or measured directly, using the equipment described in Section 3.9.1. Various studies report peak inspiratory flow rate (PIFR) values for healthy and diseased adult subjects calculated from data on inhalation without external resistance (so-called ‘control values’)(e.g. [22,100,105,106]). As for the attainable pressure drop, asthma, COPD and cystic fibrosis may decrease PIFR, depending on the severity of the disease. The addition of an external airflow resistance, such as a DPI strongly affects the PIFR.Within the range of resistances for marketed DPIs, the average PIFR at maximal inhalation by healthy adults may decrease from 159 l min–1 (for R = 0.015 kPa0.5 min l–1, equals the Rhone Poulenc Spinhaler™) to only 62 l min–1 (for R = 0.040 kPa0.5 min l–1, equals the Astra Turbuhaler™) [22]. A maximal average flow rate of approximately 60 l min–1 through the Turbuhaler has also been reported for asthmatics (e.g. references [103,107,108]).
Various flow parameters may be relevant to good DPI performance.The effect of PIFR on the in vitro dose of fine particles from DPIs has been the subject of many studies (e.g. [109–112]. The actual dose of the fine particle fraction produced varies with the ranges of flow rates applied. The flow rate being the measuring principle used for the study and definition of the fine fraction. Most studies show a strong increase in fine particle output with increasing PIFR for the ASTRA Turbuhaler™ [110–112]. For the Glaxo Diskus™ and Diskhaler™, the effect of PIFR is less extreme: in some cases an more or less constant, but also a much lower output of approximately 15 to 25% of the label claim, has been obtained [109,110]. Some recent studies refer to the fact that the Pulmicort Turbuhaler™ also has a constant fine particle yield if the acceleration towards peak flow (flow increase rate: FIR) is high enough [113,114]. For a FIR > 8 l s–2, the fine particle output is already maximal at flow rates above 40 l min–1. For capsule inhalers, the inhalation time has to be long enough for all the particles to pass rapidly through the narrow holes pierced in the capsule ends before inhalation stops.
3.9.1 Measurement of the Inspiratory Flow Curve
Many different techniques are available for flow measurement and for recording of respiratory functions or flow parameters in particular (e.g. [115,116]). However, not all methods are appropriate for measurement of inhalation flows, either because they have low frequency responses or they influence the shape of the inspiratory flow curve by a large volume or by the inertia of the measuring instrument (e.g. rotameters). They may also interfere with the aerosol cloud from the inhalation device during drug deposition studies.
78 3 Pulmonary Drug Delivery: Delivery To and Through the Lung
Electronic equivalents of traditional spirometers (pneumotachographs, pneumotachometers or anemometers) are often used in clinical practice. They integrate expiratory flow rates in order to compute flow–volume curves, from which expiratory parameters such as PEF (peak expiratory flow rate) and FEV1 (forced expiratory volume in 1 s) are derived (e.g. [22,117]). They are often laminar flow meters (e.g. Vitalograph 2100 Spirometer, Jaeger Masterscreen IOS), measuring the volumetric flow rate (Φv) at the upstream pressure of the flow head. They can also be used for measuring the inspiratory flow curve, but the flow rate has to be corrected for pressure and air density when they are used with an add-on resistance (e.g. during simulation of inhalation through dry powder inhalers) [118]. Similar corrections, including discharge and correction coefficients, are necessary for head meters (e.g. venturi or orifice meters) [119]. Thermal mass flow meters, also referred to as ‘hot-wire pneumotachographs’ do not require these corrections, but they have a high internal resistance, which makes them inappropriate for patient characterization studies. Another great disadvantage of the techniques mentioned so far is that they are in-line components of the total flow scheme, which makes them difficult to use for in vitro drug deposition studies. For this reason, on-line pressure drop measurement across a flow constriction in the flow set-up is often recommended. The flow constriction can be the add-on resistance (during patient characterization) or the inhalation device (during drug deposition measurement). Once the airflow resistance of the flow constriction is known from previous calibration (as in Table 3.7 for DPIs), the flow curve can be monitored (or adjusted) on the differential pressure signal without interfering with the aerosol cloud or adding additional resistance to the flow set-up using Eq. 3.2a for the calculations.
3.10In Vitro Particle Size Analysis and Deposition Measurements
Methods for analysis of the particle size distribution in the aerosol cloud include techniques such as time of flight measurement (TOF), inertial impaction and laser diffraction. Dynamic light scattering (photon correlation spectroscopy) is confined to particles (in suspension) in the submicron range. In addition to the size distribution, the particle velocity distribution can be measured with the Phase Doppler technique.
Inertial impaction is most widely applied for the characterization of inhalation systems. The principles of particle separation on the basis of inertial and drag forces have been well described for many different applications. Theoretical cut-off diameters (for particles with 50% collection efficiency) of impactors can be calculated on the basis of Stokes numbers for nozzles of a particular design [8,120]. Many different designs are available, but only a few are described in the United States and European Pharmacopoeia [121,122].
Inertial impaction has many inaccuracies and limitations and there are also some relevant differences between deposition in vitro (impactor) and in vivo (respiratory tract) which cause poor correlation between impactor data and lung deposition data. The most important difference is that deposition in vitro is by inertial impaction only, whereas deposition in vivo is by sedimentation and diffusional deposition as well. Except for the possible passage of the final stage (by the finest particles), particle collection in vitro is almost 100% efficient. In con-
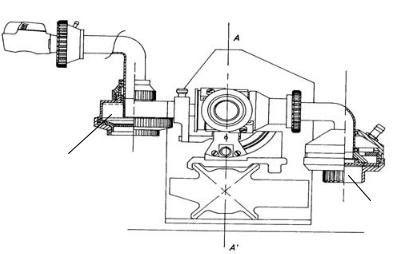
3.10 In Vitro Particle Size Analysis and Deposition Measurements |
79 |
trast, collection efficiency in vivo decreases with decreasing particle size (deposition mechanism) to a minimum of 20% for particles with an aerodynamic diameter of 0.5 m (e.g. [8]). The classification by impactors into a small number of classes for the relevant particle sizes (one to seven) may provide insufficient discrimination. For example, the theoretical cut-off (with 50% collection efficiency) for the third stage of the multistage liquid impinger (MSLI) is 3.1 m at 60 l min–1 for particles with a true density of 1.5 g cm–3. So, the fraction retained from the fourth impactor stage plus filter is smaller than 3.1 m. If the MMAD of this fraction is 2 m, deposition efficiency in the respiratory tract can be nearly 40%. If however, the MMAD of this fraction is only 1 m, the deposition efficiency is only about 20%, which is twice as low. This difference cannot be judged from the cascade impactor result.
Usually, the inspiratory flow through impactors is an on–off function: a (solenoid) valve is opened to allow suction at a pre-set flow rate through the inhalation device over a certain period. Some recent guidelines prescribe a flow rate corresponding with 4 kPa pressure drop for DPIs. Asking and Olsson derived a useful relationship for the effective cut-off diameters for the different stages of the MSLI as function of the flow rate for the range between 30 and 100 l min–1 [123]. Impactors have relatively large volumes and high airflow resistances which confine the range of adjustable PIFR and FIR. Probably the major drawback of cascade impactor analysis is that the technique is extremely laborious and time consuming.
Laser diffraction is a fast alternative for analysis of the size distribution of particles in an aerosol cloud. The theory of laser diffraction is well understood [124,125]) but this technique requires special measures to test inhalation devices and to interpret the results correctly. One of the major problems is that flow adjustment through the inhaler is not possible. Furthermore, the presence of carrier particles from adhesive mixtures may disturb the measurement of the fine drug particles and the size distribution obtained is of an unknown delivered mass fraction of the dose. These practical problems and limitations have been solved by the design of a new modular inhaler adapter for the Sympatec™ laser diffraction apparatus (Figure 3.6).
Preseparator
Fine particle collector
Figure 3.6. Schematic representation of a new inhaler adapter for laser diffraction characterization of the aerosol cloud.
80 3 Pulmonary Drug Delivery: Delivery To and Through the Lung
The adapter for DPI testing consists of a closed central housing with a pre-separator for large (carrier) particles and a fine particle collector for analysis of the fine particle mass fraction [126]. The adapter for nebulizer testing can be tilted in order to fit the angled mouthpieces of this type of inhalation device. The closed system allows accurate control of the inspiratory flow rate through the inhaler without limitations regarding PIFR and FIR.
The application of the laser diffraction technique is sometimes questioned because it measures geometric instead of aerodynamic particle diameters. However, the aerodynamic diameter can be calculated when the dynamic shape factor and density are known. Moreover, the dynamic shape factor (χ) of micronized particles will often be only slightly higher than 1 and so is the true particle density (1.0 < ρP < 1.4 g cm–3). As a consequence, the aerodynamic diameter differs only slightly from the equivalent volume diameter (see Eq. 3.1).
3.11In Vitro and In Vivo Deposition Efficacy of Inhalation Systems
Many studies present and discuss in vitro and in vivo drug deposition results obtained with inhalation systems. It is often difficult to compare in vitro results from different studies, because different testing equipment and different definitions for the fine particle dose may have been used.
The aerosol clouds from nebulizers have been investigated both with the laser diffraction technique (e.g.[48,60]) and with cascade impactors [45,49]. Droplet size distributions generated by 14 different devices (including eight jet and six ultrasonic) from laser diffraction analysis were presented by Le Brun et al. for an aqueous 10% tobramycin solution [127]. They found volume median diameters ranging from 1.3 to 3.3 µm at an inspiratory flow rate of 40 l min–1 (X90-values ranging from 2.3 to 7.9 µm). Other factors that may influence the droplet size (distribution) are surface tension and viscosity of the drug solution, nozzle pressure and inspiratory flow rate (e.g. [45,57,60]). The in vivo deposition from standard nebulizers is furthermore influenced by the patient’s breathing pattern (waste of aerosol during exhalation) and the output (rate) of the device (e.g. [47,48]). As a result of all these critical factors, lung deposition from nebulizers is generally low, between 2 and 12% of the dose for jet nebulizers [47] and between 1 and 32% for ultrasonic devices [23].
Many studies refer to the particle size (distribution) of droplets in the aerosol from MDIs (e.g. [51,128]). Droplet sizes from conventional CFC-MDIs are often not optimal for deep lung deposition: the MMADs range from approximately 2.7 to 4.8 µm [51,52,128,129].That is why in vitro fine particle (< 5 µm) fractions may be as low as 3 to 11% of the delivered dose for MDIs with the drug in solution (e.g. reference [130]) versus 20 to 35% for suspension MDIs [130,131]. Because initial particle velocities are high (exceeding 30 m s–1 at the actuator orifice), considerable losses occur in the oropharynx [23]. Under optimal conditions, including the inhalation technique, no more than 15 to 20% of the dose is deposited in the lungs (e.g. reference [29,131,132]). Newly formulated HFA(hydrofluoroalhane)-MDIs may be better, with MMADs between 1 and 2 µm [51,128,129], although it has been shown that the mean particle diameter depends on the type of HFA and increases with increasing diameter of the actuator orifice as well as increasing the concentration of non-volatile components in
3.12 Targeting Drugs to the Lungs via the Bloodstream |
81 |
the drug formulation [51]. In vitro fine particle fractions may be increased to 20–40% of the metered dose. Recently, an even higher in vitro FPF of 60% has been reported for the 3M QVAR™ Beclomethasone-HFA MDI [133], corresponding to a lung deposition of 54.1% [134].
It has been shown that the maximal in vitro FPF of most marketed devices is between approximately 20 and 50% of the nominal dose, but the maxima are achieved at different flow rates [109,110]. Lung depositions from a great number of DPI studies, summarized in two review articles [112,135], show that most systems have the same efficacy as MDIs (between 5 and 20% of the metered dose) with a few exceptions, such as the Easyhaler™, Turbuhaler™ and Novolizer™, which yield lung depositions between 20 and 30% of the dose, or even higher [135–137].
3.12 Targeting Drugs to the Lungs via the Bloodstream
This chapter mainly deals with theoretical backgrounds and strategies for the pulmonary delivery of drugs for the treatment of lung diseases, or for the administration of systemically-re- quired drugs. For the treatment of lung diseases, one may also apply drug targeting constructs via the bloodstream. As already mentioned, this is not the focus of this chapter. Yet, a few examples will be highlighted here to give a brief view on the approaches that are under investigation in this respect.
Various studies deal with gene targeting, e.g. as a treatment modality for cystic fibrosis or to induce mucosal immune responses. To determine delivery and expression efficiency, plasmids encoding reporter genes such as chloramphenicol acetyltransferase (CAT), luciferase or alkaline phosphatase are used. DODAC : DOPE (dioleoyldimethylammonium-Cl : di- oleoylphosphatidyl-ethanolamine) liposomes complexed with reporter plasmid DNA, deposited DNA in the alveolar region in the lung after i.v. administration. In comparison, intratracheal administration of the same formulation predominantly led to the deposition of DNA in epithelial cells lining the bronchioles [138]. A similar result was obtained with a formulation that consisted of DNA encoding CAT complexed with a ninth generation polyamidoamine (PAMAM) dendrimer. This polymer is a 467-kDa spherical molecule with a diameter of ~114 Å. Repeated i.v. administration allowed prolonged transgene expression [139]. Macroaggregated albumin can also be used to target plasmid DNA to the lung after i.v. injection, particles being mostly distributed into the alveolar interstitium. Using this approach, human growth hormone as an antigen-encoding plasmid elicited both mucosal and systemic immune responses in mice [140]. An important observation was the fact that inflammatory conditions significantly altered the expression of functional proteins that were systemically delivered as polycationic liposome-formulated plasmids. Although plasmid delivery per se was not affected by the disease, gene expression by the microvascular endothelium was altered to some extent [141].
Whereas all of the above-mentioned approaches are based on passive retardation of the particles in the lungs, several active targeting strategies aimed at the endothelial cells lining the pulmonary blood vessels have also been explored. Efficiency of targeting genes and the enzyme glucose oxidase, e.g. by anti-PECAM-1 antibody carriers, is based on the fact that the pulmonary vasculature contains roughly one-third of the endothelial cells in the body. Upon
82 3 Pulmonary Drug Delivery: Delivery To and Through the Lung
i.v. injection, all drug conjugates will encounter the endothelial lining of the lungs. Using a carrier consisting of a cationic polymer polyethylenimine and anti-PECAM-1 antibody, Li and colleagues were able to selectively deliver model plasmid DNA into pulmonary endothelium.This was associated with a decrease in circulating TNFα levels as compared to the levels seen with the injection of polyethylenimine/plasmid, indicating less toxic side-effects of the targeted strategy [142]. Using a similar approach with anti-PECAM-1 antibody, the enzyme glucose oxidase was selectively delivered to the pulmonary endothelium serving as a model for oxidative pulmonary vascular injury [143].
In general, when the cells of the endothelium in the lungs are the target cells of interest (see Chapters 7 and 9 on aspects of targeting drugs to endothelium in inflammatory diseases and cancer, respectively), systemic administration seems the route of choice. Bronchial epithelium on the other hand can more easily be reached via the pulmonary route. The accessibility of other cells in the lungs is most likely governed by disease conditions, factors that can affect epithelial permeability and vascular permeability, and others as described earlier.
3.13 Final Conclusions and Perspectives
Pulmonary drug administration is likely to become a rapidly growing field in drug delivery over the next two decades. Its potential to serve as a port of entry for the systemic administration of peptides and proteins makes this route an attractive choice for many of the compounds evolving from the rapidly growing field of biotechnology. However, many of the exciting possibilities that have been described over past years, lack sufficient substantiation at this time. Much experimental work is still required for many products in development before they can be introduced as a pulmonary dosage form that guarantees reproducible delivery through the lung.
Too often results are compromised by a poor experimental set-up of the studies and nontransparent data. Even essential information such as the relevant physicochemical characteristics of the drug in relation to the chosen aerosol system or the fraction that is deposited in the alveoli is often not provided. This makes it impossible to evaluate the impact of such studies. As a result, it is unclear until now to what extent and at what rate macromolecular drugs (> 20 kDa) can be absorbed by the lung. Moreover, the routes by which macromolecules pass through the different pulmonary membranes, especially the alveolar membrane, are unknown. Appropriate experiments and models that provide adequate answers to these questions are required in the coming years.
With regard to the systemic administration of smaller proteins (<20 kDa), the development of insulin for inhalation has shown that the pulmonary route is a feasible route of administration. However, advanced inhalation devices and formulations were required to obtain a reproducible lung deposition. It will be especially necessary to deal with the problems that occur when drugs with a small therapeutic window are administered. To enable widespread use of the lung as port of entry for these small proteins, future developments should be directed towards more simple inhalation devices which still give a high and reproducible lung deposition. The formulations that will be required for these proteins are likely to be much more complex and advanced than those that are currently used. Examples are formu-
References 83
lations that not only stabilize the protein, but also deliver it in the adequate physicochemical state to the absorbing membrane. In addition, it will be necessary to monitor closely the dissolution rate of drug in order to control the rate of absorption. Only if these requirements are met, will it be possible for a significant number of potential therapeutic proteins to be administered by the pulmonary route.
With regard to delivery to the lung, local therapies for asthma or COPD have an established position. New developments will focus on inhalation devices and formulations that allow a more reproducible and easy generation of the aerosol cloud and a less critical inhalation procedure. Generally, improved deposition of the drug in the airways is desirable to improve dosing accuracy and decrease side-effects. Together with the introduction of new drug substances technical improvements can still significantly improve the therapy of pulmonary diseases. For example, a major improvement in the treatment of cystic fibrosis can be achieved in the coming 5 years due to the development of new inhalation therapies for antibiotic drugs. Currently, the pulmonary use of about 10 antibiotic drugs has been reported but only two (tobramycin and colistin) have found a place in regular prophylactic use and therapy. Moreover, these drugs are still administered by quite inefficient nebulizers that provide only a deep lung deposition of less than 10% of the administered dose. Both the development of effective antibiotics against microorganisms such as Pseudomonas aeruginosa and Burkholderia cepacia as well as the development of innovative formulations and devices to improve lung deposition can largely optimize antibiotic therapy. Among others, improvements in this field can lead to better treatment of cystic fibrosis and increase life expectations. On the longer term, pulmonary administered gene therapy might even further improve cystic fibrosis therapy. Yet, significant technical hurdles have to be overcome before widespread use in therapy can be achieved.
In conclusion, it can be stated that the pulmonary administration of drugs is likely to expand rapidly in the coming years. Yet many questions still exist and extensive basic research is required before its therapeutic potential can be fully exploited in daily therapeutic practice.
Acknowledgements
Dirk K. F. Meijer is acknowledged for critically reading the manuscript. Bert Stok is thanked for his help in preparing the reference list.
References
[1]Pharmaceutical Inhalation Aerosol Technology (Ed. Hickey AJ), Marcel Dekker Inc., New York,
1992.
[2]Inhalation Delivery of Therapeutic Peptides and Proteins (Eds Adjei AL, Gupta PK), Marcel Dekker Inc., New York, 1997.
[3]Patton JS, Bukar J, Nagarajan S, Adv. Drug Deliv. Rev. 1999, 35, 235–247.
[4]Crook K, Porteous DJ, In: Inhalation Delivery of Therapeutic Peptides and Proteins (Eds Adjei AL, Gupta PK), pp. 555–585. Marcel Dekker Inc., New York, 1997.
[5]Johnson LG, Boucher RC, In: Inhalation Delivery of Therapeutic Peptides and Proteins (Eds Adjei AL, Gupta PK), pp. 515–553. Marcel Dekker Inc., New York, 1997.