
Radiation Physics for Medical Physiscists - E.B. Podgorsak
.pdf
3.2 Emission of Radiation by Accelerated Charged Particle |
99 |
Fig. 3.5. Radiation intensity distributions for two accelerated electrons; one with β = 0.006 corresponding to an electron kinetic energy of 10 eV and θmax of 89.2◦ and the other with β = 0.941 corresponding to an electron kinetic energy of 1 MeV and θmax of 10◦ . Both distributions are normalized to 1 at θmax. The actual ratio of radiation intensities at θmax = 89.2◦ and θmax = 10◦ is 1 vs. 1.44 × 104, as shown in Table 3.1
•Note that in Fig. 3.5 the maximum values of both β distributions are normalized to 1. In reality, as shown in Table 3.1, if the maximum value for the β = 0.006 distribution is 1, then, for the β = 0.941 distribution, it is more than four orders of magnitude larger at 1.44 × 104.
3.2.8 Characteristic Angle θmax
It is evident that, as β increases, the emitted radiation intensity I(r, θ) becomes more forward-peaked, and its peak intensity that occurs at a characteristic angle θmax also increases. The characteristic angle θmax is determined as follows:
Set dI(r, θ)/dθ |θ=θmax = 0, where I(r, θ) is given in (3.17), to obtain
2 sin θmax cos θmax |
− |
5β sin3 θmax |
= 0 . |
(3.18) |
(1 − β cos θmax)5 |
(1 − β cos θmax)6 |
Equation (3.18) yields a quadratic equation for cos θmax, given as follows
3β cos2 θmax + 2 cos θmax − 5β = 0 . |
(3.19) |
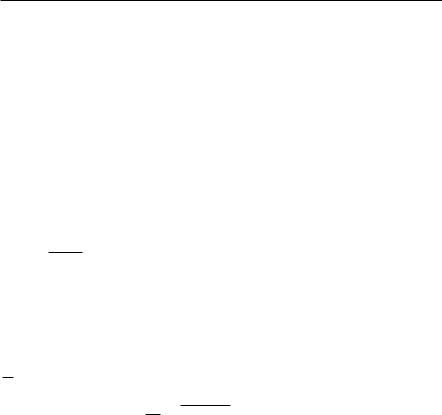
100 3 Production of X Rays
Table 3.1. Various parameters for bremsstrahlung production by electrons with kinetic energy EK
EK (MeV) |
β(a) |
γ(b) |
θmax(c) |
CI(r, θmax)(d) |
|
10−5 |
0.006 |
1.00002 |
89.2◦ |
1.0000 |
|
10−4 |
0.020 |
1.0002 |
87.2◦ |
1.0025 |
|
10−3 |
0.063 |
1.002 |
81.2◦ |
1.024 |
|
10−2 |
0.195 |
1.02 |
64.4◦ |
1.263 |
|
10−1 |
0.548 |
1.20 |
35.0◦ |
6.47 |
|
1 |
0.941 |
2.96 |
10.0◦ |
1.44 |
× 104 |
10 |
0.999 |
20.4 |
1.4◦ |
1.62 |
× 1011 |
102 |
0.9999 |
70.71 |
0.4◦ |
1.64 |
× 1015 |
|
|
|
|
|
|
(a) β = v |
= 1 |
|
|
|
1 |
|
|
|
, where mec2 |
= |
0.511 MeV for electrons and |
|||||||||||
|
|
|
|
|
|
|
|
2 |
||||||||||||||
|
c |
|
|
− 1+ |
EK |
|
|
|
|
|
|
|
|
|
|
|||||||
|
|
|
|
mec2 |
|
|
|
|
|
|
|
|
|
|||||||||
positrons |
|
|
|
|
|
|
|
|
|
|
|
|
|
|
|
|
|
|
|
|
||
1 |
|
|
|
|
|
|
|
|
|
|
|
|
|
|
|
|
|
|
|
|
||
(b) γ = |
√ |
|
|
|
|
|
|
|
|
|
|
|
|
|
|
|
|
|
|
|
|
|
1−β2 |
|
|
1 |
|
|
|
|
|
|
|
|
|
|
|
|
|
|
|
|
|||
(c) θmax = arccos |
|
|
|
1 + 15β2 − 1 , where β is given in (a) |
||||||||||||||||||
3β |
|
|
||||||||||||||||||||
|
|
|
|
sin2 |
θ |
|
|
|
2 |
a |
2 |
|
− |
1 |
||||||||
|
|
|
|
max |
|
|
e |
|
|
|
||||||||||||
(d) CI(r, θmax) = |
(1−β cos θmax)5 |
|
, where C = |
16π2εo c3r2 |
|
|
|
The physically acceptable solution of the quadratic equation is cos θmax =
31β ( 1 + 15β2 − 1), resulting in the following expression for θmax:
θmax = arccos |
1 |
( 1 + 15β2 |
− 1) . |
(3.20) |
3β |
•The limiting values for θmax of (3.20) are as follows:
–Classical region: as β → 0; θmax → π/2.
–Relativistic region: as β → 1; θmax → 0.
–In the extreme relativistic region the following approximation holds:
θmax ≈ 1/(2γ) . |
(3.21) |
•The characteristic angle θmax drops rapidly from 90◦ as β increases.
•Even for β = 0.5, corresponding to a γ of 1.155 and a relatively low electron kinetic energy of 80 keV, the characteristic angle θmax is 38.2◦.
•In the relativistic region θmax is very small being of the order of 1/2γ, i.e., of the order of the ratio of the particle’s rest energy Eo to its total energy E.
•Table 3.1 lists parameters β, γ, θmax and I(r, θmax) for bremsstrahlung production by electrons and positrons with kinetic energies between 10−5 MeV and 102 MeV.

3.2 Emission of Radiation by Accelerated Charged Particle |
101 |
Fig. 3.6. Normalized electron velocity β against the kinetic energy EK of the electron
Fig. 3.7. Characteristic angle θmax against kinetic energy EK of the electron
The entry CI(r, θmax) in Table 3.1 highlights the significant increase in the bremsstrahlung photon distribution at θ = θmax and confirms the rapid increase in x-ray production e ciency with an increase in electron (or positron) kinetic energy.
Parameters β and θmax, given in Table 3.1, are also plotted against the electron kinetic energy EK in Figs. 3.6 and 3.7, respectively. For very low kinetic energies EK (classical region) β ≈ 0 and θmax ≈ 90◦. As EK increases, β rises and asymptotically approaches 1 for very high EK, while θmax decreases with increasing EK and asymptotically approaches 0◦ for very high EK. In
the orthovoltage x-ray range θmax ≈ 40◦; in the megavoltage x-ray range
θmax ≈ 5◦.

102 3 Production of X Rays
3.3 Synchrotron Radiation
Synchrotron radiation refers to electromagnetic radiation emitted by charged particles following a curved trajectory in free space under the influence of a magnetic field. The phenomenon was first observed in 1947 in synchrotrons (hence the term synchrotron radiation); accelerators that accelerate charged particles in circular orbits to very high relativistic energies. Since the e ect occurs under the influence of a magnetic field that keeps the particles in a circular trajectory, it is sometimes called magnetic bremsstrahlung.
Electrons as well as heavier charged particles may produce the synchrotron radiation. The radiation can be considered: (1) an unnecessary nuisance causing energy losses when the objective is to attain high kinetic energies of charged particles in circular accelerators or (2) an extraordinary dedicated source of intense, short duration, x ray or ultraviolet pulses that can be exploited as a tool to study structure of matter on an atomic, molecular and cellular scale or to devise ultra fast imaging studies in cardiology. Originally, research on synchrotron radiation was conducted as a sideline to particle acceleration, recently, however, special sources of synchrotron radiation called storage rings were built with the specific purpose to produce and exploit synchrotron radiation.
The magnetic field exerts a Lorentz force on the charged particle perpendicularly to the particle’s direction of motion causing particle’s acceleration and, according to the Larmor relationship of (3.10), emission of photons. Larmor relationship of (3.10) for power P radiated by particle of charge q accelerated with acceleration a is given as follows
P = |
1 q2a2 |
. |
(3.22) |
||
6πεo |
|
c3 |
|||
|
|
|
|
For a classical particle in circular motion with radius R, the acceleration is simply the centripetal acceleration υ2/R, where υ is the velocity of the particle.
For a relativistic particle with velocity υ → c and mass m = γmo, where mo is the particle’s rest mass, in circular motion in a circular accelerator with radius R, the acceleration is similarly obtained from
|
F = moa = |
dp |
, |
(3.23) |
|
|
|||
|
dt |
|
||
where |
|
|||
p |
is the relativistic momentum of the particle: p = mυ = γmoυ, |
|
||
t |
is the proper time in the particle’s reference frame given as: t = t/γ = |
t1 − β2.
Neglecting the rate of change of γ with time t, the acceleration a can now be written as
1 |
|
dp |
|
γ d(γmoυ) |
|
2 dυ |
|
2 |
υ2 |
|
|||||
a = |
|
|
|
= |
|
|
|
= γ |
|
|
= γ |
|
|
. |
(3.24) |
|
|
|
|
|
|
|
|
|
|
||||||
mo dt |
mo |
|
dt |
|
|
dt |
|
|
R |
|

ˇ |
103 |
3.4 Cerenkov Radiation |
The power radiated from a relativistic particle according to Larmor relationship is as follows
1 |
|
q2a2 |
|
|
q2γ4 υ4 |
|
cq2β4γ4 |
|
|
||||
P = |
|
|
|
= |
|
|
|
|
= |
|
|
. |
(3.25) |
6πεo |
|
c3 |
6πεoc3 |
|
R2 |
|
6πεoR2 |
||||||
|
|
|
|
|
|
|
|
Since we know that the particle total energy E is given as E = γmoc2 = γEo, where Eo is the particle rest energy, we write (3.25) as follows
|
cq2β4 |
|
E |
4 |
|
|
P = |
|
. |
(3.26) |
|||
6πεoR2 |
Eo |
For highly relativistic particles, υ → c and the energy loss rate is governed by γ4 = (E/Eo)4 when R is fixed for a given accelerator. Equation (3.26) suggests that the larger is the accelerator radius R the smaller is the rate of energy loss.
The radiation loss ∆E during one complete revolution of a highly relativistic particle (β ≈ 1) is calculated by first determining the duration τ of one revolution as
τ = |
2 πR |
≈ |
2πR |
(3.27) |
|
|
|
. |
|||
υ |
c |
The radiation loss in one revolution is then
|
cq2 |
|
E |
|
4 |
πR |
|
q2 |
|
E |
|
4 |
|
∆E = P τ = |
|
|
|
|
|
2 |
= |
|
|
|
|
. |
(3.28) |
6πεoR2 |
Eo |
|
c |
3εoR |
Eo |
The radiation energy loss per turn is inversely proportional to the radius R of the orbit and linearly proportional to (E/Eo)4.
For electrons (q = e and mo = me = 0.511 MeV) we get the following expression for ∆E:
∆E = |
e2 |
E4 |
|
|
10−8 |
eV · m |
|
E4 |
|
|
|
|
|
|
= 8.8 |
× |
|
|
. |
(3.29) |
|||
|
3εo(mec2)4 |
|
R |
|
|
(MeV)4 |
|
R |
|
The energy is radiated in a cone centered along the instantaneous velocity of the particle. The cone has a half angle θsyn approximated as (Eo/E). For highly relativistic particles the cone is very narrow and the radiation is emitted in the forward direction similarly to the situation with the bremsstrahlung loss by relativistic particles, discussed in Sect. 3.2.8.
The wavelength distribution of synchrotron radiation follows a continuous spectrum in the x-ray, ultraviolet and visible region, with the peak emitted wavelength linearly proportional to R and (Eo/E)3.
ˇ
3.4 Cerenkov Radiation
As discussed in Sect. 3.2, a charged particle radiates energy in free space only if accelerated or decelerated; a charged particle in rectilinear uniform

104 3 Production of X Rays
velocity motion in free space does not lose any of its kinetic energy in the form of photon radiation. However, if a charged particle moves with uniform rectilinear motion through a transparent dielectric material, part of its kinetic energy is radiated in the form of electromagnetic radiation if the particle velocity υ exceeds the phase velocity of light cn in the particular medium, i.e.,
υ > cn = |
c |
|
, |
(3.30) |
|
n |
|||||
|
|
|
where n is the index of refraction of light in the particular medium.
The phenomenon of visible light emission under these conditions is re-
ˇ ˇ
ferred to as Cerenkov radiation and was discovered by Pavel A. Cerenkov and Sergei I. Vavilov in 1934.
ˇ
The emitted Cerenkov radiation does not come directly from the charged
ˇ
particle. Rather, the emission of Cerenkov radiation involves a large number of atoms of the dielectric medium that become polarized by the fast charged particle moving with uniform velocity through the medium. The orbital electrons of the polarized atoms are accelerated by the fields of the charged particle and emit radiation coherently when υ > cn = c/n.
• |
ˇ |
|
|
|
||
Cerenkov radiation is emitted along the surface of a forward directed cone |
||||||
|
centered on the charged particle direction of motion. The cone is specified |
|||||
|
with the following relationship: |
|
||||
|
cos θcer = |
cn |
= |
1 |
, |
(3.31) |
|
|
|
||||
|
|
υ |
βn |
|
ˇ
where θcer is the Cerenkov angle defined as the angle between the charged particle direction of motion and the envelope of the cone.
•Equation (3.31) suggests that there is a threshold velocity υthr below
ˇ
which no Cerenkov radiation will occur for a given charged particle and absorbing dielectric
υthr = |
c |
= cn |
(3.32) |
|
n |
||||
|
|
|
||
βthr = 1/n . |
(3.33) |
– For υ > υthr
angle θcer.
ˇ
– For υ < υthr no Cerenkov photons are produced.
ˇ
– The velocity threshold for Cerenkov radiation in water is υthr = (1/1.33)c = 0.75c.
– The velocity threshold of 0.75c for water corresponds to an energy threshold
(EK)thr = |
|
mec2 |
|
|
= |
nmec2 |
|||||
|
|
|
|
|
√ |
|
|
|
= 0.775 MeV. (3.34) |
||
|
|
|
|
|
|
|
|||||
1 − |
υthr |
|
2 |
n |
2 |
|
|||||
|
c |
|
|
|
|
− 1 |
|
3.5 Practical Considerations in Production of Radiation |
105 |
|
ˇ |
|
|
Thus, Cerenkov radiation occurs in water for electrons with kinetic |
|
|
energies exceeding 0.775 MeV. |
|
• |
ˇ |
|
Cerenkov radiation is independent of charged particle mass but depends |
on particle charge and particle velocity υ.
•Equation (3.31) also shows that there is a maximum angle of emission (θcer)max in the extreme relativistic limit where β → 1
(θcer)max = arccos(1/n) .
Thus, for relativistic electrons (β → 1) in water (n = 1.33), (θcer)max = 41.2◦.
• ˇ
Cerenkov radiation frequencies appear in the high frequency visible and near visible regions of the electromagnetic spectrum, but do not extend into the x-ray region because for x rays n < 1.
• Since the refraction index n depends on the wavelength λ of the emitted
|
ˇ |
|
radiation, the emission angle θcer for Cerenkov radiation also depends on |
|
ˇ |
|
the frequency of the Cerenkov radiation in addition to depending on the |
|
charged particle velocity υ. |
• |
ˇ |
For emission of Cerenkov radiation,2the number of quanta per wavelength |
|
|
interval ∆λ is proportional to 1/λ , favoring the blue end of the visible |
|
spectrum. This explains the characteristic bluish glow surrounding the |
|
fission core of a swimming-pool nuclear reactor or surrounding the high |
|
activity cobalt-60 sources stored in water-filled storage tanks prior to |
|
ˇ |
|
their installation in teletherapy machines. The Cerenkov radiation results |
|
from Compton electrons that propagate through water with velocities υ |
|
exceeding c/n = 0.75c. |
•As the charged particle moves through a dielectric, the total amount
ˇ
of energy appearing as Cerenkov radiation is very small compared to the total energy loss by a charged particle through collision (ionization) and radiative (bremsstrahlung) losses. For example, electrons in water lose about 2 MeV/cm through collision and radiative losses and only
ˇ
about 400 eV/cm through Cerenkov radiation losses, i.e., about a factor
ˇ
of 5000 times less. It is obvious that Cerenkov radiation is negligible as far as radiation dosimetry is concerned.
• ˇ ˇ
The Cerenkov-Vavilov e ect is used in Cerenkov detectors not only to detect fast moving charged particles but also to determine their energy
ˇ
through a measurement of the Cerenkov angle.
3.5 Practical Considerations in Production of Radiation
The sections above dealt with general classical and relativistic relationships governing the emission of radiation by accelerated charged particles, including bremsstrahlung as the most important means and two more-specialized
ˇ
phenomena: the synchrotron radiation and the Cerenkov radiation.
106 3 Production of X Rays
In principle, all charged particles can emit radiation under certain conditions. In practice, however, the choice of charged particles that can produce measurable amounts of radiation of interest in medical physics and medicine is limited to light charged particles (electrons and positrons) that can undergo the following interactions:
1.Rapid deceleration of energetic electrons in targets through inelastic Coulomb collisions of electrons with nuclei of the target resulting in superficial, orthovoltage, or megavoltage x rays (bremsstrahlung) for use in diagnosis (imaging) and treatment (radiotherapy) of disease.
2.Deceleration of electrons in retarding potentials resulting in microwave radiation. This process is used in magnetrons to produce radiofrequency photons and in klystrons to amplify radiofrequency photons. The radiofrequency used in standard clinical linear accelerators is 2856 MHz (S band); in miniature linear accelerator waveguides (tomotherapy and robotic arm mounting) it is at 104 MHz (X band).
3.Deceleration of electrons resulting in bremsstrahlung production in patients irradiated with photon or electron beams producing unwanted dose to the total body of the patient.
4.Acceleration of electrons in a linac waveguide (rectilinear motion of electrons) resulting in unwanted leakage radiation.
5.Circular motion of electrons in circular accelerators resulting in synchrotron radiation (sometimes referred to as magnetic bremsstrahlung) produced in high-energy circular accelerators and in storage rings. When charged particles pass through transverse magnetic fields, they experience an acceleration that, according to Larmor’s relationship, results in emission of radiation that is typically of lower energy than bremsstrahlung. In comparison with synchrotron radiation, the accelerations in production of bremsstrahlung are random and also much larger. Production of synchrotron radiation is still a very expensive undertaking, as it involves very expensive and sophisticated circular accelerators.
6.Deceleration of positrons (slowing down before annihilation) in positron emission tomography (PET) imaging studies of human organs resulting in unwanted stray radiation.
7.Atomic polarization e ects when electrons move through transparent dielectric materials with a uniform velocity that exceeds the speed of light
ˇ
in the dielectric material result in visible light referred to as Cerenkov
ˇ
radiation. The e ciency for production of Cerenkov radiation is several orders of magnitude lower than the e ciency for bremsstrahlung production.
8.High energy electrons striking a nucleus may precipitate nuclear reactions (e,n) or (e,p) and transform the nucleus into a radioactive state thereby activating the treatment room and also the patient.
3.6 Particle Accelerators |
107 |
3.6 Particle Accelerators
Numerous types of accelerators have been built for basic research in nuclear and high-energy physics, and most of them have been modified for at least some limited use in radiotherapy. Irrespective of the accelerator type two basic conditions must be met for particle acceleration:
1.Particle to be accelerated must be charged.
2.Electric field must be provided in the direction of particle acceleration.
The various types of accelerators di er in the way they produce the accelerating electric field and in how the field acts on the particles to be accelerated.
As far as the accelerating electric field is concerned there are two main classes of accelerators: electrostatic and cyclic.
In electrostatic accelerators the particles are accelerated by applying an electrostatic electric field through a voltage di erence, constant in time, whose value fixes the value of the final kinetic energy of the particle. Since the electrostatic fields are conservative, the kinetic energy that the particle can gain depends only on the point of departure and point of arrival and, hence, cannot be larger than the potential energy corresponding to the maximum voltage drop existing in the machine. The energy that an electrostatic accelerator can reach is limited by the discharges that occur between the high voltage terminal and the walls of the accelerator chamber when the voltage drop exceeds a certain critical value (typically 1 MV).
The electric fields used in cyclic accelerators are variable and nonconservative, associated with a variable magnetic field and resulting in some close paths along which the kinetic energy gained by the particle di ers from zero. If the particle is made to follow such a closed path many times over, one obtains a process of gradual acceleration that is not limited to the maximum voltage drop existing in the accelerator. Thus, the final kinetic energy of the particle is obtained by submitting the charged particle to the same, relatively small, potential di erence a large number of times, each cycle adding a small amount of energy to the total kinetic energy of the particle.
Examples of electrostatic accelerators used in medicine are: superficial and orthovoltage x-ray tubes and neutron generators. The best known example of a cyclic accelerator is the linear accelerator (linac); other examples are microtrons, betatrons and cyclotrons.
3.6.1 Betatron
The betatron was developed in 1940 by Donald W. Kerst as a cyclic electron accelerator for basic physics research; however, its potential for use in radiotherapy was realized soon thereafter.
•The machine consists of a magnet fed by an alternating current of frequency between 50 and 200 Hz. The electrons are made to circulate in a

108 3 Production of X Rays
Fig. 3.8. Schematic diagram of a betatron. Left: vertical cross section; right: top view
toroidal vacuum chamber (doughnut) that is placed into the gap between two magnet poles. A schematic diagram of a betatron is given in Fig. 3.8.
•Conceptually, the betatron may be considered an analog of a transformer: the primary current is the alternating current exciting the magnet and the secondary current is the electron current circulating in the vacuum chamber (doughnut).
•The electrons are accelerated by the electric field induced in the doughnut by the changing magnetic flux in the magnet; they are kept in a circular orbit by the magnetic field present in the doughnut.
•In the 1950s betatrons played an important role in megavoltage radiotherapy. However, the development of linacs pushed them into oblivion because of the numerous advantages o ered by linacs over betatrons, such as: much higher beam output (up to 10 Gy/min for linacs vs 1 Gy/min for betatrons); larger field size; full isocentric mounting; more compact design; and quieter operation.
3.6.2 Cyclotron
The cyclotron was developed in 1930 by Ernest O. Lawrence for acceleration of ions to a kinetic energy of a few MeV. Initially, the cyclotron was used for basic nuclear physics research but has later on found important medical uses in production of radionuclides for nuclear medicine as well as in production of proton and neutron beams for radiotherapy. The recent introduction of the PET/CT machines for use in radiotherapy has dramatically increased the importance of cyclotrons in medicine. The PET/CT machines rely on glucose labeled with positron-emitting fluorine-18 that is produced by proton cyclotrons.
•In a cyclotron the particles are accelerated along a spiral trajectory guided inside two evacuated half-cylindrical electrodes (referred to as “dees” because of their D-shape form) by a uniform magnetic field (1 tesla) that is produced between the pole pieces of a large magnet. A schematic diagram of the cyclotron is given in Fig. 3.9.